INTRODUCTION
The stromal vascular fraction (SVF) isolated from
adipose tissue contains adipogenic progenitors with
fibroblast-like morphologies (Van et al., 1976). These
cells have been referred to by various names including
preadipocytes and vascular stromal cells. In this
paper, we refer to the adherent stromal cells isolated
from adipose tissue as adipose-derived stromal (or
stem) cells (ASCs). Monoclonal (Zuk et al., 2002)
and polyclonal (Zuk et al., 2001) culture studies
have shown that human ASCs can be obtained from liposuction
aspirates and can differentiate into multiple lineages
of mesodermal or extodermal origin. In both in vitro
and in vivo studies, human ASCs have been shown to
differentiate not only into mesenchymal lineages such
as adipogenic, chondrogenic (Erickson et al., 2002;
Awad et al., 2003; Huang et al., 2004), osteogenic
(Halvorsen et al., 2004; Dragoo et al., 2003; Cowan
et al., 2004; Hicok et al., 2004; Peterson et al.,
2005), myogenic (Mizuno et al., 2002; Rodriguez et
al., 2005), and cardiomyogenic (Strem et al., 2005,
Planat-Benard et al., 2004a) lines but also into neurogenic
(Safford et al., 2002; Ashjian et al. 2003; Kang et
al., 2003), angiogenic (Planat-Benard et al., 2004b;
Rehman et al., 2004; Miranville et al., 2004, Cao
et al., 2005), and hepatic (Seo et al., 2005) lineages.
Liposuction is one of the most popular cosmetic surgical
procedures; worldwide, an estimated one million liposuctions
are performed annually. Although liposuction yields
a large volume (e.g., 1 L) of adipose tissue and is
considered the typical method for clinically harvesting
ASCs, liposuction aspirates have not been well researched
for use in clinical situations. Liposuction aspirates
are comprised of fatty and fluid portions (Fig. 1a).
The fatty portion consists of suctioned adipose tissue
that has been “shredded” by the reciprocal movement
of a metal canulla and vacuum pressure (500-700 mm
Hg), while the fluid portion is the liquid aspirated
along with the fatty portion. The fluid portion is
primarily composed of 1) a saline solution pre-operatively
injected into the site to prevent nerve and blood
vessel damage, 2) peripheral blood, and 3) cells or
tissue fractions derived from adipose tissue. Although
only the fatty portion of liposuction aspirates has
been investigated to date, we recognized through a
preliminary survey that a significant amount of progenitor
cells can be isolated from the liquid portion as well.
Cells isolated from the fatty portion have been termed
processed lipoaspirate (PLA) cells (Zuk et al., 2001;
Zuk et al., 2002), and here we refer to cells isolated
from the fluid portion as liposuction aspirate fluid
(LAF) cells.
ASCs are currently being used in clinical trials including
studies investigating bone defect (Lendeckel et al.,
2004) and rectovaginal fistula (Garcia-Olmo et al.,
2005) treatments and soft tissue augmentation (our
unpublished data). ASCs can be used clinically without
cell expansion because a sufficient number can be
obtained directly by processing liposuction aspirates,
which are usually of a large volume. Furthermore,
the use of minimally manipulated fresh cells may lead
to higher safety and efficacy in actual treatments.
Thus, because freshly isolated ASCs are preferable
to cultured ones for cell-based therapies, especially
in the initial stage of regenerative medicine, minimally
manipulated SVF from adipose tissue must be characterized
in more detail. The SVF is known to be comprised of
a heterogeneous cell population, but the exact cell
composition remains to be determined.
In this study, the fatty and fluid portions of liposuction
aspirates were investigated as sources for ASCs. Cells
isolated from both portions were characterized under
fresh and cultured conditions. In addition, long-term
changes in cell surface marker expression profiles
were determined for both cell populations.
MATERIALS AND METHODS
Human tissue sampling
After informed consent, we obtained liposuction aspirates
using an IRB-approved protocol from healthy female
donors aged 21 to 59 years who underwent liposuction
of the abdomen or thighs. Liposuction aspirates were
divided into two portions: a floating adipose portion
(also called lipoaspirate) and a denser fluid portion
(Fig. 1a). Both portions were used as sources for
PLA and LAF cells. For harvesting human vascular endothelial
cells (HUVEC) and dermal fibroblasts, umbilical cords
and skin were obtained from separate donors under
informed consent. Human mesenchymal stem cells derived
from bone marrow (frozen at passage 2) were purchased
from Cambrex Bio Science Walkersville, Inc. (NJ) and
cultured with the same medium used for adipose-derived
cells.
Cell isolation
All chemicals were purchased from Wako Pure Chemicals
(Osaka, Japan), unless otherwise stated.
PLA cells were separated from the fatty portions of
liposuction aspirates using a procedure modified from
Zuk et al. (2001). Briefly, the suctioned fat was
digested with 0.075% collagenase in PBS for 30 min
on a shaker at 37oC. Mature adipocytes and connective
tissues were separated from pellets by centrifugation
(800 x g, 10 min). Pellets were resuspended in erythrocyte
lysis buffer (155mM NH4Cl, 10mM KHCO3, 0.1mM EDTA)
and incubated for 5 min at room temperature. The pellets
were resuspended and passed through a 100-μm mesh
filter (Millipore, MA, USA).
LAF cells were harvested from the fluid portions of
liposuction aspirates. The suctioned fluid was centrifuged
(400 x g, 10 min), and the pellets were resuspended
in erythrocyte lysis buffer. After 5 min at room temperature,
lysates were passed through a 100-μm mesh filter.
The pellets were then processed for density gradient
centrifugation with Ficoll (Amersham Biosciences,
NJ, USA). After centrifugation (800 x g, 20 min),
cells at the gradient interface were collected, washed
with PBS, and passed through a 100-μm mesh filter.
For flow cytometry of freshly isolated LAF cells,
density gradient centrifugation was not conducted.
Nucleated cell counts were performed using a NucleoCounter
(Chemometec, Denmark).
Cell culture of adherent cells from adipose tissue
Freshly isolated PLA or LAF cells were plated in medium
at a density of 5 x 106 nucleated cells/100-mm gelatin-coated
dish. Cells were cultured at 37oC, 5% CO2, in humid
air. The culture medium was M-199 containing 10% FBS,
100 IU penicillin, 100 mg/ml streptomycin, 5 ng/ml
heparin, and 2 ng/ml acidic FGF. For measurement of
doubling time, the same medium was used with a serum
concentration of 10% or 15%. Primary cells were cultured
for 7 days and were defined as “Passage 0.” The medium
was replaced every 3 days, and cells were passaged
every week. After primary culture for 7 days, attached
cells were passaged by trypsinization and plated in
the same medium at a density of 2,000 cells/cm2.
Induced differentiation
of cultured PLA and LAF cells
Capacities to differentiate along adipogenic, chondrogenic,
and osteogenic lineages were examined. Seven days
after seeding PLA or LAF cells at passage 3-5, cell
differentiation was initiated by replacing the M-199
culture medium. Cells cultured in control medium (DMEM
plus 100 IU penicillin and 100 mg/ml streptomycin)
containing 10% FBS were used as negative controls.
For adipogenic differentiation, confluent cultures
were incubated for 4 weeks in the control medium containing
10% FBS supplemented with 0.5 mM isobutyl-methylxanthine
(Sigma, MO), 1 μM dexamethasone, 10 μM insulin (Sigma),
and 200 μM indomethacin. Fixed cells (4% paraformaldehyde
for 10 min) were washed with 60% isopropanol and incubated
for 15 min with Oil-Red O to visualize lipid droplets.
Cells were then washed with isopropanol and counterstained
with hematoxylin.
For chondrogenic differentiation, two types of evaluations
were performed. First, confluent cultures were incubated
for 4 weeks with the control medium containing 1%
FBS supplemented with 6.25 μg/ml insulin, 10 ng/ml
TGFβ1, and 50 nM ascorbate-2-phosphate. Fixed cells
(4% paraformaldehyde for 10 min) were washed with
3% acetic acid and incubated for 30 min with 1% Alcian
Blue 8 GX (Sigma), 3% acetic acid to visualize the
extracellular matrix. Cells were then washed with
3% acetic acid and counterstained with 0.1% Nuclear
Fast Red, 5 % Al2(SO4)3 solution. Second, a micromass
culture system was used as previously reported (Johnstone
et al., 1998). Cells were pelleted in a 15-ml tube
and cultured with the chondrogenic medium for 3 weeks.
For osteogenic differentiation, cells were incubated
for 4 weeks in the control medium containing 10% FBS
supplemented with 0.1 μM dexamethasone, 50 μM ascorbate-2-phosphate,
and 10 mM β-glycerophosphate (Nacalai Tesque, Kyoto,
Japan). Fixed cells (4% paraformaldehyde for 10 min)
were washed and incubated with 2.5% silver nitrate
for 20 min in the dark. The cells were then washed,
placed in the light for 15 min, and incubated for
2 min with 0.5% hydroquinone. Cells were incubated
for 2 min with 5% sodium thiosulphate to visualize
calcified deposits. The cells were then washed and
counterstained with 0.1% Nuclear Fast Red, 5% Al2(SO4)3
solution.
For adipogenic differentiation, colony-forming unit
analysis was also performed to quantify colonies with
intracellular lipids. One hundred fifty cells were
plated on a 100-mm culture disk and cultured for 10-14
days, followed by incubation for 2 weeks with adipogenic
medium and staining with Oil-Red O as described above.
The numbers of colonies positive or negative for staining
were counted under a microscope.
Flow cytometry and sorting
Freshly isolated PLA and LAF cells were examined for
surface and intracellular molecule expression using
flow cytometry. In addition, adherent PLA and LAF
cells were examined at weeks 1, 2, 4, 6, 8, 10, and
20 of cell culture. The following monoclonal antibodies
(MAbs) conjugated to fluorochromes were used: anti-CD4-FITC,
CD10-PE, CD13-PE, CD16-PE, CD29-PE, CD31-PE, CD34-PE,
CD34-FITC, CD34-PE Cy7, CD36-PE, CD44-PE, CD45-PE,
CD45-FITC, CD49d-PE, CD49e-PE, CD54-PE, CD56-PE, CD57-FITC,
CD62E-PE, CD62P-PE, CD69-FITC, CD73-PE, CD90-PE, CD106-FITC,
CD117-PE, CD135-PE, CD146-PE, CD-151-PE, HLA-A,B,C-PE,
Tie-2-PE (BD Biosciences, San Diego, CA, USA), CD31-APC
(eBioscience, CA, USA), CD144-PE (Beckman Coulter,
CA, USA), CD59-PE (Ancell, Bayport, MN, USA), CD71-PE,
CD105-PE (Serotec, Oxford, UK), CD133-PE, and Flk-1-PE
(Techne, NJ, USA). Irrelevant control MAbs were included
for all fluorochromes. Cells were incubated with directly
conjugated MAbs for 30 min, then washed and fixed
in 1% paraformaldehyde. Cells were analyzed using
a LSR II (Becton Dickinson, San Jose, CA, USA) or
FACS Vantage SE (Becton Dickinson) flow cytometry
system. Data acquisition and analysis were then performed
(Cell Quest software, Becton Dickinson). Gates were
set based on staining with combinations of relevant
and irrelevant MAbs so that no more than 0.1% of cells
were positive using irrelevant antibodies. Cell sorting
and subsequent analyses were performed using a FACSAria
cell sorter (Becton Dickinson).
Statistical Analyses
Results were expressed as mean ± SEM. Welch's t-test
was used to compare each parameter.
RESULTS
Isolation and expansion of stromal cells from fatty
and fluid portions of liposuction aspirates
Compared to the fatty portion of liposuction aspirates,
the fluid portion contains more peripheral blood discharged
from the suctioned site during liposuction. The volume
of peripheral blood varied among patients. The adipose
portion was subjected to collagenase digestion followed
by filtration for exclusion of extracellular matrix
(ECM) fragments and debris, while the fluid portion
was centrifuged and processed for lysis of contaminating
erythrocytes. After plating on culture dishes, non-adherent
cells were discarded by changing the culture medium.
Both adherent PLA and LAF cells had fibroblast-like
morphologies and proliferated with similar doubling
times, although a smaller number of adherent cells
were harvested from LAF cells than from PLA cells
(Fig. 1b).
Cell yields were normalized by dividing the isolated
cell number by the volume (in liters) of the fatty
portion of the liposuction aspirate. Normalized numbers
of nucleate cells in SVF from the adipose (fresh PLA
cells; n=28) and fluid (fresh LAF cells; n=28) portions
were 1.31 ± 0.50 x109 and 1.55 ± 0.79 x109 per 1 L
of adipose portion (P=0.401), respectively (Fig.1c).
However, the cell number varied considerably among
patients. Erythrocyte contamination was seen in both
fresh PLA and LAF cells, although LAF cells apparently
contained a much greater number of cells derived from
peripheral blood. After 1 week of cell culture, normalized
numbers of adherent PLA (n=28) and LAF (n=28) cells
were 9.7 ± 1.7 x107 and 3.0 ± 0.6 x107 (P<0.001),
respectively (Fig.1d). Thus, there was no significant
difference in cell yield between freshly isolated
PLA and LAF cells, but there was a difference between
adherent PLA and LAF cells cultured for 1 week.
PLA and LAF cells were cultured in medium with 10%
(n=14) or 15% FBS (n=15), and doubling times were
measured using cells at passage 0. Doubling times
of PLA and LAF cells were 28.5 ± 1.7 h and 31.0 ±
2.6 h, respectively, when cultured with 15% FBS, and
43.3 ± 2.8 h and 40.2 ± 3.0 h, respectively, when
cultured with 10% FBS. A statistically significant
difference in doubling time was observed between the
two serum concentrations in both PLA (P<0.001)
and LAF (P<0.05) cells, but not between PLA and
LAF cells at either serum concentration (Fig.1e).
In vitro differentiation
of PLA and LAF cells
To compare the multipotency of PLA and LAF cells,
cell differentiation was induced using cells at passage
3-5 by culturing the cells for 4 weeks with adipogenic,
chondrogenic, or osteogenic medium. The results showed
that both cell populations have similar capacities
to differentiate along the adipogenic (Fig. 2a), chondrogenic
(Fig. 2b), and osteogenic lineages (Fig. 2c). In addition,
similar cartilage formation was observed by the micromass
system (Fig. 2d). Colony-forming unit analysis showed
that the percentage of cells staining positive for
Oil-Red O was 29.0 ± 7.6% for PLA cells and 24.1 ±
4.3% for LAF cells (P=0.12) (Fig. 2d).
Flow cytometric analysis
of PLA and LAF cells
Flow cytometric analysis revealed that freshly isolated
LAF cells differ significantly in cell surface marker
expression from freshly isolated PLA cells. Compared
to fresh LAF cells, fresh PLA cells contained higher
percentages of cells positive for CD29 (β1-integrin),
CD34, and CD90 (Thy-1) expression and a decreased
percentage of CD45+ cells of hematopoietic origin.
Conversely, in fresh LAF cells, there were higher
percentages of CD31 (PECAM-1)+ cells and CD45+ cells,
suggesting that fresh LAF cells contained a larger
number of blood-derived cells than fresh PLA cells.
In the FACS plot of forward and side scatter characteristics
(FSC and SSC) of fresh LAF cells, there were three
CD45+ cell clusters corresponding to granulocytes,
monocytes/macrophages, and lymphocytes (Figs. 3a,
S1). CD34+ cells and CD45- cells were located in the
cluster corresponding to monocytes/macrophages (Figs.
3a, S1). Double color analysis of fresh LAF cells
for CD34 and CD45 indicated that most of the CD34+
cells were CD45-, suggesting that most of the CD34+
cells were not derived from peripheral blood but from
adipose tissue (Fig. 3b). In addition, CD34+CD45-
cells, but not CD34+CD45+ cells, proliferated on a
culture dish, suggesting that the freshly isolated
LAF cells contained adherent stromal cells derived
from adipose tissue (Fig. 3b). CD34+ cells (P2) were
sorted and cultured using the protocol described above,
but after 2 weeks in culture approximately half of
the cells were negative for CD34 (Fig. 3b), suggesting
that some CD34+ cells lost CD34 expression with increased
culture time. The CD34- cells did not reacquire CD34
expression by further culturing. The doubling time
of CD34- cells was significantly shorter than that
of CD34+ cells (Fig. 3c).
Subsequently, we performed multicolor FACS assays
to investigate the SVF (fresh PLA and LAF cells) in
more detail, especially with regard to cell composition.
Even after processing with hypotonic erythrocyte lysis
buffer, the SVF contained a large number of erythrocytes,
so erythrocytes and platelets were excluded from analysis
by gating them out by cell size. Consequently, only
nucleated cells were analyzed (Figs. 4 and 5). The
results are summarized in Table 1. We classified freshly
isolated cells derived from liposuction aspirates
into 11 cell populations [4 blood-derived (CD45+)
and 7 adipose-derived (CD45-)] as follows. There were
three major (> 1%) and one minor (< 1%) cell
populations derived from blood with regard to expression
patterns of CD31, CD34, CD45, CD105 (Endoglin), CD14,
and CD15 (Figs. 5a, S2): 1) CD31lowCD34-CD45+CD105lowCD14-CD15+
cells (corresponding to granulocytes), 2) CD31lowCD34-CD45+CD105lowCD14+CD15-
cells (corresponding to monocytes/macrophages), 3)
CD31-CD34-CD45+CD105- cells (corresponding to lymphocytes),
and 4) CD31-CD34+CD45+CD105- cells (corresponding
to hematopoietic stem cells). Cell compositions varied
among samples, with the percentages of the first three
populations dependent on the composition of the peripheral
blood of each sample. Furthermore, the total percentage
of blood-derived populations in SVF depends on the
intraoperative hemorrhage volume of each sample.
There were seven adipose-derived populations with
regard to expression patterns of CD31, CD34, CD45,
CD90, CD105, and CD146: 1) CD31-CD34+CD45-CD90+CD105-CD146-
cells (corresponding to ASCs), 2) CD31+CD34+CD45-CD90+CD105low
CD146+ cells, 3) CD31-CD34+CD45-CD90+CD105-CD146+
cells, 4) CD31-CD34-CD45-CD90+CD105-CD146+ cells,
5) CD31-CD34+CD45-CD90+CD105low cells, 6) CD31lowCD34+CD45-CD90+CD105lowCD146+
cells,
and 7) CD31-CD34-CD45-CD90+CD105-CD146- cells. The
first population corresponded to ASCs and comprised
70-90% of the total adipose-derived (CD45-) cells.
Most ASCs were CD29+, CD117 (c-kit)-, CD133/AC133-,
CD144 (VE-cadherin)-, and Flk-1(VEGFR-2)- (Fig. 4a).
The second population was positive for both CD31 and
CD146 (identified as P1 in Fig. 5a; also identified
in Figs. 4b and 5b), suggesting that it is composed
of vascular endothelial cells or endothelial progenitor
cells derived from adipose. The third (CD34+) and
fourth (CD34-) populations were CD31-CD146+ (Figs.
4a and 4b), suggesting that they may correspond to
pericyte progenitors (CD34+) and pericytes (CD34-),
respectively. The fifth population was very small
and seemed to correspond to CD105-positive ASCs (P1
in Fig. 5b). The sixth population was also small,
with moderate expression of CD31 (P2 in Fig. 5a).
Finally, the seventh population was positive only
for CD90, suggesting that it may be fibroblasts or
ASCs that lost CD34 expression (P3 in Fig. 5a).
Additionally, a multi-color FACS assay was performed
for cultured PLA cells (at 5 and 10 days; Fig. 6a,
6b) and cultured BM-MSCs (passage 3; Fig. 6c) for
comparison. Most PLA cells cultured for 5 days were
CD31-CD34+CD45-CD90+CD105+CD146-, but small percentages
of other cell populations such as CD31+CD34+CD45-CD90+CD105+CD146+
cells (endothelial cells or endothelial progenitors),
CD31-CD34-CD45-CD90+CD105+CD146+ cells (possibly pericyte
progenitors), and CD31-CD34-CD45-CD90+CD105+CD146+
cells (pericytes) were also observed. Unlike freshly
isolated ASCs, the major ASC population expressed
CD105. Compared to PLA cells cultured for 5 days,
the 10-day cultures showed decreased percentages of
CD31+ endothelial cells and CD34+ cells and increased
percentages of CD31-CD34+CD146+ cells and CD31-CD34-CD146+
cells. Cultured BM-MSCs showed similar surface marker
expression patterns. Most cultured BM-MSCs were identified
as CD31-CD34-CD45-CD90+CD105+ cells with variable
expression of CD146. BM-MSCs contained only a very
small percentage (< 1%) of CD34+ cells, which was
the only difference from ASCs clearly detected in
this assay.
Representative single-color FACS data for freshly
isolated and cultured (at 2 weeks) PLA and LAF cells
are shown in Fig. 7, and sequential changes in cell
surface marker expression are shown in Fig. 8. After
plating, the surface marker expression profiles for
both PLA and LAF cells changed markedly, and adherent
cells of the two cell populations showed quite similar
expression profiles. The percentage of CD34+ cells
increased in PLA cells, which uniformly expressed
mesenchymal markers such as CD13, CD29, CD44, CD73,
and CD90. In PLA cells cultured for more than 1 week,
CD10, CD49e, CD59, and CD151 were also uniformly expressed.
One-week culture of freshly isolated PLA or LAF cells
resulted in a dramatic enrichment in CD105 from 1.2
± 0.6 to 64.1 ± 9.7 (P<0.001) or from 1.4 ± 0.7
to 74.6 ± 7.6 (P<0.001), respectively. No statistically
significant difference in CD105 expression was observed
between adherent PLA and LAF cells. After 1 week in
culture, CD45, Flk-1, Tie-2, CD31, CD117, and CD133/AC133
expression had decreased significantly in PLA and
LAF cells. Both cell populations were negative for
CD4, CD45, CD62E (E selectin), CD62P (P selectin),
CD69, CD135, and CD144 at 1 week and were negative
for CD16, CD31, CD57, CD106 (VCAM-1), CD133, Flk-1,
and Tie-2 after culture for more than 2 weeks.
CD34 expression decreased with increased culture time,
but 10-20% of cells maintained CD34 expression up
to 20 and 10 weeks’ culture in PLA and LAF cells,
respectively. In addition, consistent expression of
the mesenchymal markers (CD13, CD29, CD44, CD73, and
CD90) as well as other markers (CD 49d, CD59, CD105,
and CD151) was observed in adherent PLA and LAF cells
up to 20 and 10 weeks, respectively. After the initial
2 weeks, no remarkable changes in surface marker expression
were seen between adherent PLA and LAF cells throughout
the culture periods. Taken together, these data suggest
that adherent PLA and LAF cells can be expanded using
our culture method without losing stem cell-associated
surface markers. Our studies further revealed that
after the initial 1-2 weeks in culture, adherent PLA
and LAF cells have quite similar surface marker expression
profiles throughout the culture periods, suggesting
that both cell populations can be considered ASCs.
DISCUSSION
Adipose tissue is comprised predominantly of mature
adipocytes, connective tissue, ASCs, blood-derived
cells, vascular cells such as endothelial (progenitor)
cells, smooth muscle cells, and pericytes. Adipocytes
represent roughly two-thirds of the total cell number
and more than 90% of the tissue volume (van Harmelen
et al., 2005). The ratio of adipocytes to ASCs is
constant in humans, independent of Body Mass Index
(BMI) and age (van Harmelen et al, 2003). In the present
study, a correlation between ASC cell yield and age
or BMI was not detected (data not shown). ASC cell
yield varies among patients and is affected by many
factors including donor site and storage duration.
It also depends strongly on the isolation method,
e.g., duration of collagenase digestion (Bakker et
al, 2004; Aust et al., 2004; von Heimburg et al.,
2004).
Although only the adipose portion of liposuction aspirates
has been used as a source of ASCs, we also isolated
cells from the fluid portion of liposuction aspirates
and found that a comparable amount of adherent stromal
cells can be harvested. By flow cytometric analysis,
fresh PLA and LAF cells showed distinct surface marker
profiles. Higher percentages of CD31+ and CD45+ cells
were observed in fresh LAF cells than in PLA cells,
suggesting that fresh LAF cells contain a larger amount
of blood-derived cells. However, adherent LAF cells
cultured for one week showed surface marker profiles
quite similar to cultured PLA cells, suggesting that
the fluid portion of liposuction aspirates also contains
ASCs. In addition, our results showed that most of
the CD34+ cells in freshly isolated LAF cells were
derived from adipose tissue (CD45-), and these CD34+CD45-
cells expanded in culture dishes, suggesting that
they correspond to the same ASC population derived
from PLA cells. Why a significant amount of ASCs are
isolated from the fluid portion of liposuction aspirates
has yet to be determined. Nor is the location of ASCs
in adipose tissue clearly understood. Some ASCs are
thought to be located in the adipose connective tissue
and others between adipocytes or around micro- or
macro-vasculature. ASCs located between adipocytes
might be released into the fluid by mechanical injury
during liposuction procedures, and other ASCs might
be released by endogenous proteases during surgery
or subsequent storage periods. The extent of mechanical
injury may vary among patients because it can be affected
by the size of the suction canulla, vacuum pressure
strength during liposuction, suction procedure (manual
or powered), and other factors.
One of the reasons why adipose tissue is thought to
be a promising source of stem cells is that a large
volume of adipose tissue can be harvested with minimal
morbidity. Thus, ASCs can be used clinically after
minimal manipulation (without cell culture). Therefore,
examination and characterization of freshly isolated
PLA and LAF cells have significant clinical implications.
Some recent studies examined freshly isolated SVF
from human adipose tissue using magnetic cell sorting
(Boquest et al., 2005; Sengenes et al, 2005), and
the investigators partly characterized some cell populations
in the SVF. CD31-CD34+CD45-CD105+ cells were proposed
as typical proliferating ASCs (Boquest et al., 2005).
Other studies showed that CD34+CD31- cells differentiated
into endothelial cells and contributed to neovascularization
(Planat-Bernard et al., 2004b; Miranville et al, 2004).
For analysis of SVF, we used multicolor (4 or 5 colors)
flow cytometry assays and showed that there are several
major and minor cell populations derived from blood
and adipose. The typical surface marker expression
pattern of fresh ASCs was CD31-CD34+CD45-CD90+CD105-CD146-,
which was distinct from that of cultured ASCs in CD105
expression only. This profile of fresh ASCs differs
from a previous study (Boquest et al., 2005), possibly
because the magnetic cell sorting procedure used in
that study might have affected CD105 expression. Our
results also showed that most fresh ASCs are CD29+,
CD90+, CD117-, CD133-, CD144-, and Flk-1-. It should
be noted that there are significant numbers of CD146+
and CD146- cells in both CD34+ and CD34- cell populations
in SVF. Given that CD31 is an endothelial cell marker
and that CD146 is a known marker of endothelial cells
and pericytes, CD31-CD34-CD146+ cells in SVF are likely
pericytes, and CD31-CD34+CD146+ cells may function
as pericyte progenitors. Nearly all adipose-derived
CD31+ cells in SVF were found to be CD34+, and this
population was previously reported as endothelial
cells (Sengenes et al., 2005). Our results showed
that this population was CD90+CD105lowCD146+. However,
a major proportion of cultured HUVECs (passage 2)
were CD34- and CD90- (Fig. S3b), suggesting that fresh
but not cultured endothelial cells express CD34 and
CD90. Indeed, freshly isolated HUVEC cells were CD34+
with variable expression of CD90 (Fig. S3a). CD31-CD34-CD45-CD90+CD105-CD146-
cells in SVF may be fibroblasts, as suggested by comparison
with cultured dermal fibroblasts (Fig. S3c).
PLA and LAF cells showed similar surface marker profiles
after being cultured for one week. Notable changes
between fresh and cultured states include decreased
expression of CD31 and CD45 and increased expression
of CD29 and CD105. These changes suggest that cells
other than ASCs, such as vascular endothelial cells
and blood-derived cells, are selectively excluded
during culturing on plastic plates. Even when cultured
in endothelial cell growth medium, ASCs quickly outgrow
CD31+ endothelial cells (Hutley et al, 2001). As noted
above, a high percentage of ASCs began to express
CD105 after plating. Our results showed that the expression
of most surface markers is sustained throughout the
culture period (up to 20 weeks for PLA and 10 weeks
for LAF cells), and that significant changes seen
after the initial 2 weeks are limited to a gradual
increase in CD49d and a decrease in CD71.
Because CD34 is one of the most well established stem
cell markers, CD34 expression may indicate ASC clinical
usefulness. CD105, known as a mesenchymal stem cell-associated
marker, was highly expressed in cultured ASCs and
may reflect the capacity of ASCs to differentiate
into lineages of mesenchymal origin such as adipose,
cartilage, and bone. Flk-1, known to be expressed
in hemangioblasts, was recently shown to be expressed
in ASCs under certain culture conditions (Martinez-Estrada
et al, 2005; Cao et al., 2005). These factors suggest
that ASCs may have clinical potential for cell-based
therapies. A number of studies characterizing human
ASCs have reported that ASCs (freshly isolated or
cultured for less than 2 weeks) are CD34+ (Gronthos
et al., 2001; Planat-Bernard et al., 2004b; Rehman
et al., 2004; Miranville et al., 2004; Boquest et
al., 2005). However, CD34 expression was not found
in human ASCs cultured for more than 2 weeks with
conventional culture methods (Gronthos et al., 2001;
Zuk et al., 2002; De Ugarte et al., 2003; Rehman et
al., 2004; Lee et al., 2004; Katz et al., 2005; Boquest
et al., 2005). In contrast, our results showed that
CD34+ ASCs were present at 10-20% of the cell population
even after 10-20 weeks of culture (Fig. 7). CD34+
cells were sorted and cultured, but about half of
the cells became CD34- after 2 weeks in culture (Fig.
3b), suggesting that cultured ASCs may exist in a
variety of stages ranging from CD34+ undifferentiated
cells to CD34- partially differentiated cells. The
result that CD34+ cells proliferated more quickly
than CD34- cells may explain the sustained percentage
of CD34+ cells in cultured PLA or LAF cells. Thus,
using our culture protocol it is possible to expand
CD34+ ASCs taken from liposuction aspirates up to
104-107 times after 4 weeks’ culture. Together with
the fact that Flk-1+ ASCs can be obtained in high
percentages using another culture protocol (Martinez-Estrada
et al, 2005; Cao et al., 2005), ASCs may dramatically
change their surface marker profiles depending on
the culture media and methods.
It was reported that human ASCs differ from human
BM-MSCs in expression of CD49d (expressed only in
ASCs) and CD106 (expressed only in BM-MSCs) (Zuk et
al., 2002; De Ugarte et al., 2003). In our study using
multicolor assays with limited surface markers, the
only difference between cultured ASCs and cultured
BM-MSCs was CD34 expression (positive in ASCs and
negative in BM-MSCs). Human dermal fibroblasts have
a surface marker expression profile similar to ASCs
but lack expression of CD34 and CD105 (Fig. S3c).
Because BM-MSCs (Zuk et al., 2002; De Ugarte et al.,
2003) and other stromal progenitors cultured for long
periods do not express CD34, ASCs may constitute a
unique mesenchymal cell population in view of their
CD34 and CD146 expression. This characteristic may
contribute to potentialities of ASCs other than mesenchymal
progenitors, such as endothelial (Planat-Benard et
al., 2004b; Rehman et al., 2004; Miranville et al.,
2004) or pericyte progenitors.
In summary, adherent LAF cells have quite similar
characteristics with respect to growth kinetics, morphology,
surface marker profiles, and capacity for differentiation
to adherent PLA cells. A significant amount of ASCs
can be isolated from the fluid portion of liposuction
aspirates, although in smaller amounts than from the
fatty portion. In addition, we found that SVF are
composed of heterogeneous cell populations including
blood-derived cells, ASCs, endothelial (progenitor)
cells, pericytes (and progenitors), and other unknown
progenitors. A major population of ASCs in SVF was
identified as CD31-CD34+CD45-CD90+CD105-CD146- cells
but began to express CD105 after plating. Adipose-derived
CD34+ ASCs can be expanded for at least 20 weeks using
our culture method. These results suggest that liposuction-derived
human ASCs may have significant clinical utility for
cell-based therapies.
Figure Legends
Fig. 1. Isolation of adipose-derived stromal cells
(ASCs) from the fatty and fluid portions of liposuction
aspirates. (a) The two portions of liposuction aspirates
in a bottle. The fatty portion floats above the denser
fluid portion. Cells isolated from the fatty portion
were named PLA cells; those isolated from the liquid
portion were termed LAF cells. (b) Primary cultures
of freshly isolated PLA and LAF cells. Scale bar =
100 μm. (c) Cell yields of freshly isolated PLA and
LAF cells. No statistically significant difference
in yield was detected. (d) Adherent cell yields of
cultured PLA and LAF cells at 1 week. A significantly
higher yield of adherent PLA cells was detected. ??
* P<0.05. (e) Doubling times of adherent PLA and
LAF cells. A statistically significant difference
was seen between the two serum concentrations (10%
and 15%) in both PLA and LAF cells, but not between
PLA and LAF cells at either serum concentration. ?
* P<0.05
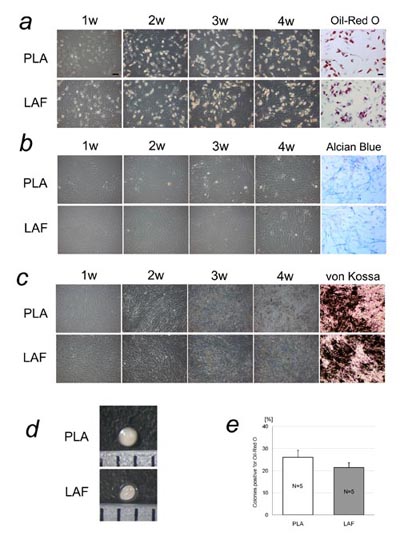
Fig. 2 Cell differentiation analysis of adherent PLA
and LAF cells. Representative results are shown in
(a)-(d). PLA and LAF cells at passage 3-5 were similarly
induced to differentiate into adipogenic, chondrogenic,
and osteogenic lineages. (a) Cultures under adipogenic
conditions. Adipogenic differentiation was induced,
and lipid droplets were visualized with Oil-Red O
staining at 4 weeks. (b) Cultures under chondrogenic
conditions. Chondrogenic differentiation was induced
and visualized with Alcian blue staining at 4 weeks.
(c) Cultures under osteogenic conditions. Osteogenic
differentiation was induced and visualized with von
Kossa staining at 4 weeks. Scale bar = 100 μm. (d)
Micromass culture of PLA and LAF cells. Cartilage
formation was similarly observed using the micromass
system. (e) Colony-forming unit analysis under adipogenic
conditions. Between adherent PLA and LAF cells, the
difference in percentages of colonies that differentiated
into an adipogenic lineage was not statistically significant.
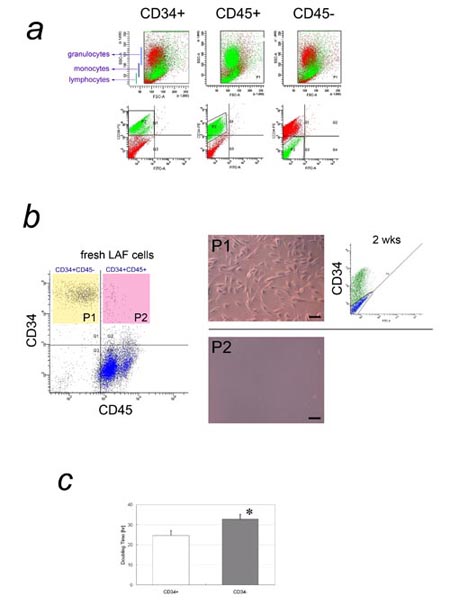
Fig. 3. CD34 expression in freshly isolated and adherent
LAF cells.
a) Flow cytometric analysis of freshly isolated LAF
cells for cell size, granularity, and expression of
CD34 or CD45. In the FACS plot of SSC (granularity)
vs. FSC (cell size) for freshly isolated LAF cells,
CD34+ cells (left) and CD45- cells (right) are shown
in green. Both were located in the same area as monocytes/macrophages,
while CD45+ cells (middle) were located in the areas
typical for blood cells such as granulocytes, monocytes/macrophages,
and lymphocytes (see Fig. S1). b) Double color flow
cytometric cell sorting of fresh LAF cells by CD34
and CD45 expression, and the fates of sorted cells
after plating. Left: Most CD34+ cells from fresh LAF
cells were CD45-. Middle: The CD34+CD45- (P1) and
CD34+CD45+ (P2) cell populations were sorted and plated;
only CD34+CD45- cells grew on the dish. Scale bar
= 100 μm. Right: After 2 weeks’ culture, approximately
half of the CD34+CD45- cells had lost CD34 expression.
c) Comparison of doubling time between CD34+ and CD34-
adherent LAF cells. The doubling time of CD34+ adherent
LAF cells was significantly shorter than that of CD34-
cells. * P<0.05
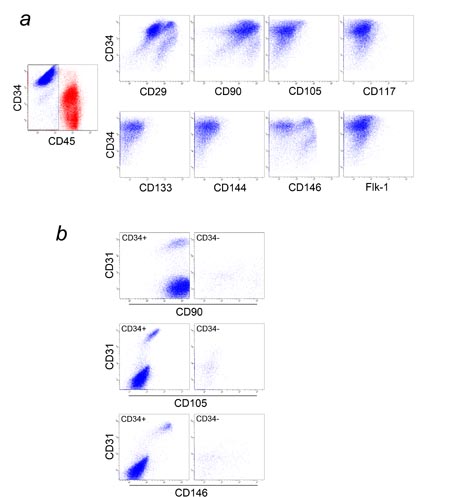
Fig. 4. Multicolor FACS analysis of SVF (1). (a) SVF
from the adipose portion of liposuction aspirates
was analyzed for CD34, CD45, and one of the following
markers: CD29, CD90, CD105, CD117, CD133, CD144, CD146,
or Flk-1. CD34 and CD45 expression is shown in the
graph at left, with blood-derived CD45+ cells in red
and adipose-derived CD45- cells in blue. Only adipose-derived
CD45- cells (blue dots) were plotted in each of the
8 graphs at right. Most adipose-derived CD34+ cells
were CD29+, CD90+, CD105-, CD117-, CD133-, CD144-,
and Flk-1-. It should be noted that there are two
populations of adipose-derived CD34+ cells with regard
to CD146 expression. (b) SVF from the adipose portion
was analyzed for CD31, CD34, CD45, and one of the
following markers: CD90, CD105, or CD146. Only adipose-derived
CD45- cells were plotted. There are two major and
one minor populations of CD34+ cells. One major population
(endothelial cells or endothelial progenitors) was
CD31+CD34+CD90+CD105lowCD146+ and comprised 2-8% of
CD34+ cells. The largest population (ASCs) was CD31-CD34+CD90+CD105-CD146-.
The minor population was CD31-CD34+CD90+CD105-CD146+.
There were two small populations of CD34- cells; one
was CD31-CD34-CD90+CD105-CD146+, and the other was
CD31-CD34-CD90+CD105-CD146-.
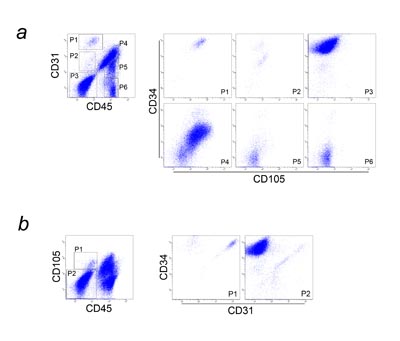
Fig. 5. Multicolor flow cytometric analysis of SVF
(2). Freshly isolated SVF from the adipose portion
was analyzed with multicolor flow cytometry for CD31,
CD34, CD45, and CD105.
(a) CD31 and CD45 expression is shown in the graph
at left, with six major populations (P1-P6) identified.
Each population was individually plotted for CD34
and CD105 expression in the six graphs at right. P1
was composed of CD31+CD34+CD45-CD105low cells, which
are fresh endothelial cells or their progenitors.
P2 was an unknown minor population (CD31lowCD34-CD45-CD105low
cells; possibly CD31low endothelial cells). P3 contained
ASCs, which are CD31-CD34+CD45-CD105-. P4 consisted
predominantly of granulocytes and monocytes/macrophages,
with a small number of lymphocytes. P5 and P6 consisted
of lymphocytes (Fig. S2).
(b) CD45 and CD105 expression is shown in the graph
at left, and two adipose-derived (CD45-) populations
(P1: CD34+, P2: CD34-) are identified. Each population
was individually plotted for CD31 and CD34 expression
in the graphs at right. P1 was comprised of CD31+CD34+CD45-CD105low
cells (fresh endothelial cells or progenitors) and
CD31-CD34+CD45-CD105low cells. P2 was composed of
other adipose-derived cells including ASCs.
Fig. 6. Multicolor FACS analysis of cultured PLA cells
and cultured BM-MSCs. PLA cells cultured for 5 days
(a) and 10 days (b) and BM-MSC (passage 3) (c) were
analyzed for CD31, CD34, CD45, and one of following
markers: CD90, CD105, or CD146.
(a), (b) In the graphs at far left, all cells were
plotted, while only CD45- cells (blue dots) are shown
in the six graphs at right. There are several differences
in surface marker expressions between freshly isolated
and cultured PLA cells; Almost all ASCs started expressing
CD105 after plating, and some ASCs lost CD34 expression
with increased culture time. CD31+ endothelial cells
decreased in percentage with culture time, while CD31-CD34-CD146+
cells (possibly pericyte progenitors) and CD31-CD34-CD146+
cells (pericytes) increased. CD31+CD90+ endothelial
cells observed in fresh SVF lost CD90 expression with
increased culture time, as seen in HUVEC (Figs. S3a
and S3b).
(c) Nearly all BM-MSCs were CD34-. Red and blue dots
are CD34+ and CD34- cells, respectively. Cultured
BM-MSCs and ASCs showed similar expression of CD31,
CD45, CD90, CD105, and CD146, with the only detectable
difference being in CD34 expression.
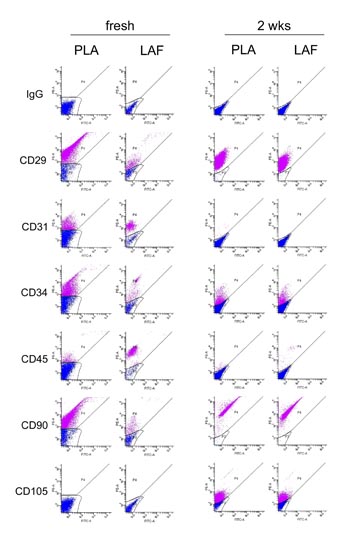
Fig. 7. Comparison of flow cytometric data for freshly
isolated and 2-week-cultured PLA and LAF cells. Freshly
isolated PLA and LAF cells displayed distinct cell
surface marker profiles, while PLA and LAF cells cultured
for 2 weeks showed quite similar expression profiles.
Adherent, but not freshly isolated, PLA and LAF cells
expressed CD105. Results are representative of samples
from five patients.
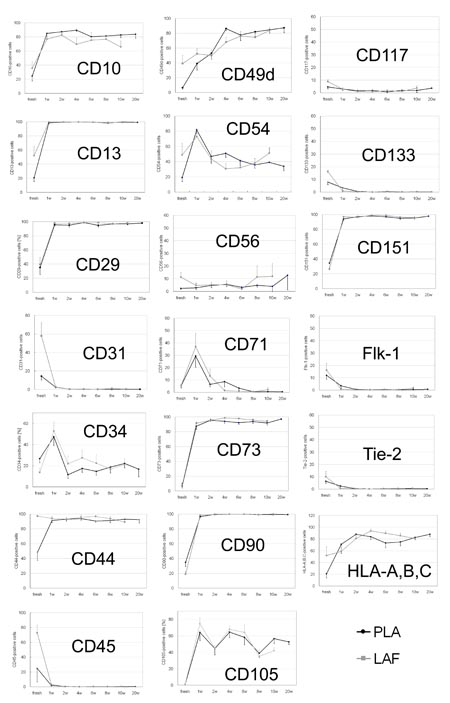
Fig. 8. Sequential changes in representative cell
surface marker expression in fresh and cultured PLA
and LAF cells. Statistically significant differences
in expression of some cell surface markers (data not
shown for CD16 and CD49e) were observed between freshly
isolated PLA and LAF cells. These differences were
not apparent after 1-2 weeks in culture. Data are
shown as mean + (LAF) or ? (PLA) standard error.
Fig. S1. FSC and SSC analysis of peripheral blood.
FACS analysis of peripheral blood by FSC (cell size)
and SSC (granularity). Blue dots are cells positive
for each CD antigen: CD4 (helper T lymphocytes), CD8
(cytotoxic T lymphocytes), CD11b (both granulocytes
and monocytes/macrophages), CD14 (monocytes/macrophages),
CD15 (granulocytes), and CD19 (B lymphocytes). Granulocytes,
monocytes/macrophages, and lymphocytes are located
predominantly in the upper, middle, and lower populations,
respectively.
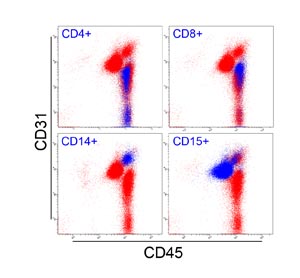
Fig. S2. Multicolor FACS analysis of peripheral blood.
Blue dots are cells positive for CD4 (helper T lymphocytes),
CD8 (cytotoxic T lymphocytes), CD14 (monocytes/macrophages),
and CD15 (granulocytes). All blood-derived cells were
CD45+. Granulocytes and monocytes/macrophages were
CD31+, while lymphocytes were CD31-.
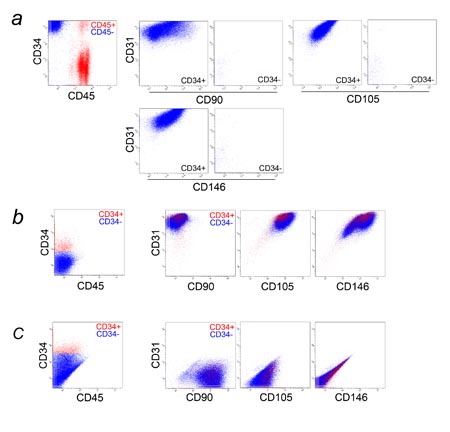
Fig. S3. Multicolor FACS analysis of freshly isolated
HUVEC, cultured HUVEC, and cultured dermal fibroblasts.
Freshly isolated HUVEC (a), cultured HUVEC (EGM-2;
passage 2) (b), and cultured dermal fibroblasts (DMEM+10%FBS,
passage 4) (c) were analyzed for CD31, CD34, CD45,
and one of following markers: CD90, CD105, or CD146.
(a) All freshly isolated cells from human umbilical
cord vein were plotted in the graph at far left, showing
contamination by blood-derived CD45+ cells (red dots).
Only adipose-derived CD45- cells (blue dots) were
shown in the six graphs at right. Most freshly isolated
HUVEC were CD31+CD34+CD45-CD105+CD146+, while CD90
expression varied.
(b) Most cultured HUVEC (passage 2) were CD31+CD34-CD45-CD90-CD105+CD146+.
Small percentages of CD31+CD34+CD45-CD90-CD105+CD146+
and CD31+CD34-CD45-CD90+CD105+CD146+ cells were also
detected. Red and blue dots are CD34+ and CD34- cells,
respectively.
(c) Most of the cultured dermal fibroblasts were CD31-CD34-CD45-CD90+CD105-CD146-,
and a small percentage was CD31-CD34+CD45-CD90+CD105-CD146-.
Red and blue dots are CD34+ and CD34- cells, respectively,
gated in the graph at far left.
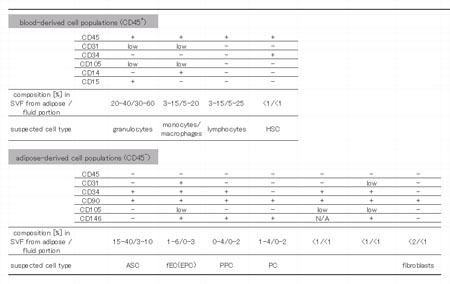
Table 1. Summary of cell composition in SVF derived
from liposuction aspirates. Results of multi-color
FACS assays are summarized. We classified freshly
isolated cells from liposuction aspirates into 11
populations according to surface marker expression
profiles; 4 blood-derived (CD45+; above) and 7 adipose-derived
(CD45-; below) cell populations. Cell composition
percentages varied among samples, so a range of values
is presented. HSC: hematopoietic stem cell, ASC: adipose-derived
stromal cell, fEC: fresh endothelial cell, EPC: endothelial
progenitor cell, PC: pericytes, PPC: pericyte progenitor
cell.
References
Ashjian PH, Elbarbary AS, Edmonds B, DeUgarte D, Zhu
M, Zuk PA, Lorenz HP, Benhaim P, Hedrick MH. 2003.
In vitro differentiation of human processed lipoaspirate
cells into early neural progenitors. Plast Reconstr
Surg 111:1922-1931.
Aust L, Devlin B, Foster SJ, Halvorsen YD, Hicok K,
du Laney T, Sen A, Willingmyre GD, Gimble JM. 2004.
Yield of human adipose-derived adult stem cells from
liposuction aspirates. Cytotherapy 6:7-14.
Awad HA, Halvorsen YD, Gimble JM, Guilak F. 2003.
Effects of transforming growth factor beta1 and dexamethasone
on the growth and chondrogenic differentiation of
adipose-derived stromal cells. Tissue Eng 9:1301-1312.
Bakker AH, Van Dielen FM, Greve JW, Adam JA, Buurman
WA. 2004. Preadipocyte number in omental and subcutaneous
adipose tissue of obese individuals. Obes Res 12:488-498.
Boquest AC, Shahdadfar A, Fronsdal K, Sigurjonsson
O, Tunheim SH, Collas P, Brinchmann JE. 2005. Isolation
and transcription profiling of purified uncultured
human stromal stem cells: alteration of gene expression
following in vitro cell culture. Mol Biol Cell 16:
1131-1141.
Cao Y, Sun Z, Liao L, Meng Y, Han Q, Zhao RC. 2005.
Human adipose tissue-derived stem cells differentiate
into endothelial cells in vitro and improve postnatal
neovascularization in vivo. Biochem Biophys Res Commun.
332:370-9.
Cowan CM, Shi YY, Aalami OO, Chou YF, Mari C, Thomas
R, Quarto N, Contag CH, Wu B, Longaker MT. 2004. Adipose-derived
adult stromal cells heal critical-size mouse calvarial
defects. Nat Biotechnol 22:560-567.
De Ugarte DA, Alfonso Z, Zuk PA, Elbarbary A, Zhu
M, Ashjian P, Benhaim P, Hedrick MH, Fraser JK. 2003.
Differential expression of stem cell mobilization-associated
molecules on multi-lineage cells from adipose tissue
and bone marrow. Immunology Letters 89:267-270.
Dragoo JL, Samimi B, Zhu M, Hame SL, Thomas BJ, Lieberman
JR, Hedrick MH, Benhaim P. 2003. Tissue-engineered
cartilage and bone using stem cells from human infrapatellar
fat pads. J Bone Joint Surg Br 85:740-747.
Erickson GR, Gimble JM, Franklin DM, Rice HE, Awad
H, Guilak F. 2002. Chondrogenic potential of adipose
tissue-derived stromal cells in vitro and in vivo.
Biochem Biophys Res Commun 290:763-769.
Garcia-Olmo D, Garcia-Arranz M, Herreros D, Pascual
I, Peiro C, Rodriguez-Montes JA. 2005. A phase I clinical
trial of the treatment of Crohn's fistula by adipose
mesenchymal stem cell transplantation. Dis Colon Rectum
48:1416-1423.
Gronthos S, Franklin DM, Leddy HA, Robey PG, Storms
RW, Gimble JM. 2001. Surface protein characterization
of human adipose tissue-derived stromal cells. J Cell
Physiol 189:54-63.
Halvorsen YC, Wilkison WO, Gimble JM. 2004. Adipose-derived
stromal cells--their utility and potential in bone
formation. Int J Obes Relat Metab Disord 24 Suppl
4:S41-44.
Hicok KC, Du Laney TV, Zhou YS, Halvorsen YD, Hitt
DC, Cooper LF, Gimble JM. 2004. Human adipose-derived
adult stem cells produce osteoid in vivo. Tissue Eng
10:371-380.
Huang JI, Zuk PA, Jones NF, Zhu M, Lorenz HP, Hedrick
MH, Benhaim P. 2004. Chondrogenic potential of multipotential
cells from human adipose tissue. Plast Reconstr Surg
113:585-594.
Hutley LJ, Herington AC, Shurety W, Cheung C, Vesey
DA, Cameron DP, Prins JB. 2001. Human adipose tissue
endothelial cells promote preadipocyte proliferation.
Am J Physiol Endocrinol Metab 281:E1037-1044.
Johnstone B, Hering TM, Caplan AI, Goldberg VM, Yoo
JU. 1998. In vitro chondrogenesis of bone marrow-derived
mesenchymal progenitor cells. Exp Cell Res 238:265?272
Kang SK, Lee DH, Bae YC, Kim HK, Baik SY, Jung JS.
2003. Improvement of neurological deficits by intracerebral
transplantation of human adipose tissue-derived stromal
cells after cerebral ischemia in rats. Exp Neurol
183:355-366.
Katz AJ, Tholpady A, Tholpady SS, Shang H, Ogle RC.
2005. Cell surface and transcriptional characterization
of human adipose-derived adherent stromal (hADAS)
cells. Stem Cells 23:412-423.
Lee RH, Kim B, Choi I, Kim H, Choi HS, Suh K, Bae
YC, Jung JS. 2004. Characterization and expression
analysis of mesenchymal stem cells from human bone
marrow and adipose tissue. Cell Physiol Biochem 14:311-324.
Lendeckel S, Jodicke A, Christophis P, Heidinger K,
Wolff J, Fraser JK, Hedrick MH, Berthold L, Howaldt
HP. 2004. Autologous stem cells (adipose) and fibrin
glue used to treat widespread traumatic calvarial
defects: case report. J Craniomaxillofac Surg 32:370-373.
Martinez-Estrada OM, Munoz-Santos Y, Julve J, Reina
M, Vilaro S. 2005. Human adipose tissue as a source
of Flk-1+ cells: new method of differentiation and
expansion. Cardiovasc Res 65:328-333.
Miranville A, Heeschen C, Sengenes C, Curat CA, Busse
R, Bouloumie A. 2004. Improvement of postnatal neovascularization
by human adipose tissue-derived stem cells. Circulation
110:349-355.
Mizuno H, Zuk PA, Zhu M, Lorenz HP, Benhaim P, Hedrick
MH. 2002. Myogenic differentiation by human processed
lipoaspirate cells. Plast Reconstr Surg 109:199-209.
Planat-Benard V, Menard C, Andre M, Puceat M, Perez
A, Garcia-Verdugo JM, Penicaud L, Casteilla L. 2004a.
Spontaneous cardiomyocyte differentiation from adipose
tissue stroma cells. Circ Res 94:223-229.
Planat-Benard V, Silvestre JS, Cousin B, Andre M,
Nibbelink M, Tamarat R, Clergue M, Manneville C, Saillan-Barreau
C, Duriez M, Tedgui A, Levy B, Penicaud L, Casteilla
L. 2004b. Plasticity of human adipose lineage cells
toward endothelial cells: physiological and therapeutic
perspectives. Circulation 109:656-663.
Peterson B, Zhang J, Iglesias R, Kabo M, Hedrick M,
Benhaim P, Lieberman JR. 2005. Healing of critically
sized femoral defects, using genetically modified
mesenchymal stem cells from human adipose tissue.
Tissue Eng 11:120-129.
Rehman J, Traktuev D, Li J, Merfeld-Clauss S, Temm-Grove
CJ, Bovenkerk JE, Pell CL, Johnstone BH, Considine
RV, March KL. 2004. Secretion of angiogenic and antiapoptotic
factors by human adipose stromal cells. Circulation
109:1292-1298.
Rodriguez AM, Pisani D, Dechesne CA, Turc-Carel C,
Kurzenne JY, Wdziekonski B, Villageois A, Bagnis C,
Breittmayer JP, Groux H, Ailhaud G, Dani C. 2005.
Transplantation of a multipotent cell population from
human adipose tissue induces dystrophin expression
in the immunocompetent mdx mouse. J Exp Med 201:1397-1405.
Safford KM, Hicok KC, Safford SD, Halvorsen YD, Wilkison
WO, Gimble JM, Rice HE. 2002. Neurogenic differentiation
of murine and human adipose-derived stromal cells.
Biochem Biophys Res Commun 294:371-379.
Sengenes C, Lolmede K, Zakaroff-Girard A, Busse R,
Bouloumie A. 2005. Preadipocytes in the human subcutaneous
adipose tissue display distinct features from the
adult mesenchymal and hematopoietic stem cells. J
Cell Physiol 205:114-122.
Seo MJ, Suh SY, Bae YC, Jung JS. 2005. Differentiation
of human adipose stromal cells into hepatic lineage
in vitro and in vivo. Biochem Biophys Res Commun 328:258-264.
Strem BM, Zhu M, Alfonso Z, Daniels EJ, Schreiber
R, Begyui R, Maclellan WR, Hedrick MH, Fraser JK.
2005. Expression of cardiomyocytic markers on adipose
tissue-derived cells in a murine model of acute myocardial
injury. Cytotherapy 7:282-291.
van Harmelen V, Skurk T, Hauner H. 2005. Primary culture
and differentiation of human adipocyte precursor cells.
Methods Mol Med 107:125-135.
van Harmelen V, Skurk T, Rohrig K, Lee YM, Halbleib
M, Aprath-Husmann I, Hauner H. 2003. Effect of BMI
and age on adipose tissue cellularity and differentiation
capacity in women. Int J Obes Relat Metab Disord 27:889-895.
Van RL, Bayliss CE, Roncari DA. 1976. Cytological
and enzymological characterization of adult human
adipocyte precursors in culture. J Clin Invest 58:699-704.
von Heimburg D, Hemmrich K, Haydarlioglu S, Staiger
H, Pallua N. 2004. Comparison of viable cell yield
from excised versus aspirated adipose tissue. Cells
Tissues Organs 178:87-92.
Zuk PA, Zhu M, Ashjian P, De Ugarte DA, Huang JI,
Mizuno H, Alfonso ZC, Fraser JK, Benhaim P, Hedrick
MH. 2002. Human adipose tissue is a source of multipotent
stem cells. Mol Biol Cell 13:4279-4295.
Zuk PA, Zhu M, Mizuno H, Huang J, Futrell JW, Katz
AJ, Benhaim P, Lorenz HP, Hedrick MH. 2001. Multilineage
cells from human adipose tissue: implications for
cell-based therapies. Tissue Eng 7:211-228.
|