Introduction
It has been revealed that adipose-derived stromal
(stem) cells (ASC) can be obtained from liposuction
aspirates1,2 and differentiate into multiple lineages
of mesodermal or ectodermal origins. Human ASCs,
which are also called pre-adipocytes or vascular
stromal cells, were shown by a number of in vitro
and in vivo studies to differentiate into adipogenic,
osteogenic3-7, chondrogenic8-10, myogenic11,12,
cardiomyogenic13,14, and neurogenic15-17 lineages.
Adipose tissue is known to be rich in microvasculature18,
and ASCs were shown to have angiogenic characteristics
and to differentiate into vascular endothelial cells
experimentally19-21. Human ASCs are distinct from
other mesenchymal progenitors in surface marker
expression profile; notably only ASCs express stem-cell?associated
marker CD34 in higher percentages compared to bone-marrow?derived
mesenchymal stem cells and dermal fibroblasts22.
ASCs are currently being used in clinical trials,
including studies investigating bone defect23 (fresh
ASCs) and rectovaginal fistula24 (cultured ASCs)
treatments and soft tissue augmentation (our unpublished
data; fresh ASCs). If ASCs are harvested from a
large volume (e.g. 1 L) of liposuction aspirates,
ASCs can be used clinically without cell expansion
because a sufficient number can be obtained. Furthermore,
the use of minimally manipulated fresh cells may
lead to higher safety and efficacy in actual treatments.
Aspirated fat is also used as injection material
for soft tissue augmentation for the purpose of
reconstruction of inborn or acquired tissue defects
or cosmetic treatments such as breast enhancement
or facial rejuvenation. Although lipoinjection has
some problems to be resolved, such as unpredictability
and a low survival rate due to partial necrosis25,26,
it is almost the only method of soft tissue augmentation
that can be performed without detectable scarring
on either a donor or a recipient site and without
complications associated with foreign materials.
Thus, if the clinical efficacy and safety of the
procedure can be improved, it could be a strong
cosmetic and reconstructive tool for soft tissue
augmentation.
Here, we provide evidence to support a novel method
of autologous tissue transfer, which we named cell-assisted
lipotransfer (CAL). CAL is a concurrent transplantation
of aspirated fat and ASCs; in other words, it is
a transplantation of ASC-rich aspirated fat. In
CAL, ASCs were supportively used to boost the efficacy
of autologous lipoinjection, meaning a higher survival
rate and persistency of transplanted fat, and to
decrease known adverse effects of lipoinjection
such as formation of fibrosis, pseudo-cyst, and
calcification. In this study, aspirated fat was
compared to excised whole fat (non-aspirated fat)
in micro- and electromicroscopic histology and adherent
cell yields. In addition, we evaluated the effectiveness
of CAL in animal models compared to non?cell-assisted
lipotransfer (non-CAL), and examined the fate of
ASCs in fat transplanted with the CAL method.
Materials and Methods
Human Tissue Sampling
We obtained liposuction aspirates from healthy female
donors undergoing liposuction of the abdomen or
thighs after informed consent using an IRB-approved
protocol. From 3 patients who underwent both liposuction
and tummy tuck, an excised adipose tissue (20?30
g) was also taken. Aspirated fat was used as the
cell source of SVFs containing ASCs. The excised
fat obtained from tummy-tuck patients was also used
for isolation of SVFs. Both the aspirated fat and
the excised fat were also used for histological
examination.
Cell isolation and culture
SVF was isolated from the fatty portion of liposuction
aspirates using a procedure modified from Zuk and
colleagues1. Briefly, the aspirated fat was washed
with PBS and digested on a shaker at 37 oC in PBS
containing 0.075% collagenase for 30 min. Mature
adipocytes and connective tissues were separated
from pellets by centrifugation (800 ~ g, 10 min).
The pellets were resuspended and filtered with a
100-Κm mesh (Millipore, MA, USA). Freshly isolated
SVF was plated (30,000 cells/cm2) on gelatin-coated
dishes and cultured at 37 oC in an atmosphere of
5% CO2 in humid air. The culture medium was M-199
containing 10% FBS, 100 IU penicillin, 100 mg/mL
streptomycin, 5 ng/mL heparin, and 2 ng/mL acidic
FGF. After 7 days, attached cells were passaged
by trypsinization and cultured in the same medium.
Medium was replaced every third day. The excised
fat from tummy-tuck patients was first minced with
scissors into small pieces (3 mm) and then processed
in the same manner as aspirated fat.
Mouse models for transplantation
of human aspirated fat
Seven-week-old male SCID mice housed with free access
to water and standard chow diet were anesthetized
by intraperitoneal injection of 5 mg/mL isopentane.
Human aspirated fat (1 mL = 900 mg) was subcutaneously
injected into the back of SCID mouse with or without
freshly isolated SVF cells. For CAL, SVFs taken
from 4 mL of aspirated fat was mixed with 1 mL of
aspirated fat. Four weeks later, transplanted fat
was harvested, weighed, and fixed with 4% paraformaldehyde,
and 4-Κm sections were stained with hematoxylin?eosin.
Mouse models for tracing human
SVF cells in CAL
For tracing the SVFs, SVF cells freshly isolated
from human aspirated fat were labeled by incubated
with 5 Κg/mL CM-DiI (Molecular Probe, Eugene, Oregon)
for 1 hour before transplantation. DiI-labeled SVFs
taken from 4 mL of aspirated fat was mixed with
1 mL of aspirated fat and then injected under the
back skin of a SCID mouse. In the same manner, human
aspirated fat was injected without DiI-labeled SVFs
as control. Four weeks later, transplanted fat was
harvested, fixed with 4% paraformaldehyde, and embedded
in OCT compound by freezing in isopentene cooled
with liquid nitrogen. Then, 20-Κm thick frozen sections
were examined with a confocal microscope system
(Leica TCS SP2, Leica Microsystems GmbH, Wetzlar,
Germany).
Rat models for tracing rat GFP-labeled
SVF in CAL using rat minced fat
Inguinal adipose tissue excised from GFP rats [SD
TgN(act-EGFP)OsbCZ-004; generated from Sprague-Dawley
(SD) rats] was processed in the same way as human
excised fat. GFP-SVF taken from 1 mL of fat of the
GFP rat was mixed with minced inguinal adipose tissue
(1 mL) harvested from SD rats and then injected
under the back skin of an SD rat. Four weeks later,
transplanted fat was harvested and forwarded to
immunohistology as well.
Immunostaining
Frozen sections were prepared from transplanted
fat embedded in OCT compound. After fixation in
100% acetone for 10 min at 4 C, sections were rehydrated
and blocked with 0.5% goat serum in PBS. Paraffin-embedded
sections were dewaxed, washed in PBS, and treated
with Proteinase K (Dakocytomation, Carpinteria,
CA) for 6 min and blocked with 0.5% goat serum in
PBS. Primary and secondary antibody stainings were
performed for 60 min each at room temperature. DAPI
staining was performed with DAPI containing mounting
medium (Vector laboratories, Burlingame, CA). The
following antibodies and dilutions were used: anti-Green
Fluorescent Protein (mouse, 1:500; Molecular Probes,
Eugene, Oregon), von Willebrand factor (rabbit,
1:500; DakoCytomation), and Alexa Fluor 488 or Alexa
Fluor 546 conjugated anti-mouse or anti-rabbit antibodies
(goat, 1:200; Molecular Probes). Sections were imaged
on a confocal microscope system (Leica TCS SP2,
Leica Microsystems GmbH).
Scanning electron microscopic study
Aspirated and excised fat were fixed with 2% paraformaldehyde
and 2.5% glutaraldehyde in 0.2 M cacodylate buffer
for a week at room temperature, and then fixed in
1% osmium tetroxide. After dehydration, they were
dried with a super critical point CO2 dryer (HCP-2,
Hitachi, Tokyo, Japan), sputter-coated with Pt-Pd,
and examined with a scanning electron microscope
(S3500N, Hitachi).
Statistical analyses
Results were expressed as mean } standard error.
The data were statistically analyzed using an unpaired
Studentfs t-test. A value of p<0.05 was considered
significant.
Results
Comparison in histology and ASC
yield between aspirated fat and excised fat
Both aspirated fat and excised fat were harvested
from the 3 patients who underwent the tummy-tuck
operation. Histological examinations with a light
microscope and scanning electron microscope showed
that aspirated fat preserves basic structures of
adipose tissue and mature adipocytes keep normal
adhesions with each others. The only difference
detected was that aspirated fat contained fewer
vascular structures, especially those of large size,
compared to excised fat.
The same weights of aspirated fat and excised fat
were processed for isolation of SVFs. The cell isolation
process was performed within two hours after harvest.
The isolated SVFs were cultured for 1 week, and
the numbers of adherent ASCs from the aspirated
fat and the excised fat were counted and compared.
In all 3 patients, the number of adherent ASCs at
1 week was larger in the excised fat than in the
aspirated fat. The ratio of normalized ASC number
from aspirated fat to that from excised fat was
0.48 } 0.13 (n=3, mean } S.E.) (Fig. 1b).
Human aspirated fat transplantation
with or without SVF
Human aspirated fat was transplanted with or without
freshly isolated SVFs containing ASCs taken from
the same patient. The experiments were done three
times using aspirated fat from three patients.
Transplanted adipose tissue with or without ASCs
(CAL fat and non-CAL at) was 712.3 } 45.3 mg (n
= 10) or 520.6 } 40.8 mg (n = 11), respectively,
while fresh adipose tissue (1 mL) before transplantation
was approximately 900 mg (Fig. 2a, 2b). Histology
of transplanted fat samples showed that the central
region was necrotic in non-CAL fat samples and the
thickness of survived layer was greater in CAL fat
than non-CAL fat (Fig. 2c). Microvasculature microscopically
detected appeared to be prominent in CAL fat, especially
in the outer layers, but not in non-CAL fat (Fig.
2c).
Fate of human ASCs transplanted
with human aspirated fat
To trace human ASCs, SVFs freshly isolated from
aspirated fat were labeled with DiI and then transplanted
with human aspirated fat. In CAL fat, DiI-labeled
ASCs were occasionally detected between mature adipocytes
and in the connective tissue of the transplanted
fat (Fig. 3). ASCs double-positive for DiI and vWF
were detected in CAL fat, suggesting that some of
transplanted ASCs were differentiated into vascular
endothelial cells (Fig. 4).
Fate of ASCs derived from GFP rats
in rat CAL models
To trace ASCs transplanted with fat, minced fat
of SD rats was transplanted with (CAL) or without
(non-CAL) SVFs isolated from the inguinal adipose
of GFP rats (GFP-SVF) (Fig. 5a). In CAL fat, GFP-positive
cells, which are supposed to be transplanted GFP-ASCs,
were detected within connective tissue and in some
vessels (Fig. 5b). Most of the vessels were derived
from host (vWF-positive, GFP-negative), but vessels
positive for both vWF and GFP were occasionally
detected. GFP-positive cells covered partly or entirely
the inner surface of vessels (Fig. 5b). It was suggested
that some ASCs differentiated into endothelial cells
and contributed to angiogenesis during the surviving
process of the adipose transplantation.
Discussion
Adipose tissue is predominantly composed of extracellular
matrix, mature adipocytes, ASCs, vascular cells
such as endothelial cells, and mural cells such
as pericytes and vascular smooth muscle cells. We
recently reported the cell composition of SVFs freshly
isolated from liposuction aspirates, which is important
information for the clinical use of the SVFs. The
SVF cells are composed of heterogeneous populations
including blood-derived cells, which are composed
of 50%-70% SVF cells. Adipose-derived cells (CD45-
cells) in SVFs isolated from aspirated fat were
of the following types: ASCs (70%-90%; CD31-CD34+CD45-CD90+CD105-CD146-),
vascular endothelial (progenitor) cells (3%-9%;
CD31+CD34+CD45-CD90+CD105low CD146+), pericytes
(2%-5%; CD31-CD34-CD45-CD90+CD105-CD146+), and other
cells. The cell composition of the SVFs was measured
after the cell isolation process with collagenase
digestion for 30 min, but not all of adipose-derived
cells can be isolated from the SVFs in this process;
thus, the cell composition may not accurately correspond
to the actual cell composition of the adipose tissue.
The location of ASCs in the adipose tissue is not
clearly understood. Some ASCs are supposed to be
located in the connective tissues in adipose, and
others are located between adipocytes or around
micro- or macrovasculature.
Liposuction aspirates consist of two parts: a floating
fatty portion and an infranatant fluid portion.
We have investigated cells derived from these two
portions of liposuction aspirates and found that
a significant number of adipose-derived cells, including
ASCs and endothelial cells, can be isolated from
the fluid portion, though the number from the fluid
portion is smaller than that from the fatty portion.
This finding supports a result of this study showing
that aspirated fat is relatively stem-cell deficient
compared to excised whole fat. The reason was not
elucidated here, but mechanical injury during the
liposuction procedure and digestion by endogenous
proteases during the surgery or subsequent storage
periods likely induces the release of ASCs from
harvested aspirated fat into the fluid portion.
In addition, as shown in this study, the basic structure
of adipose tissue was preserved in the aspirated
fat, but vascular vessels, especially larger ones,
are significantly less detected in aspirated fat
compared to excised fat. It is well known that the
honeycomb structures of vascular and neural perforator
networks are left intact in aspirated sites after
liposuction operation (Fig. S1). Thus, it is reasonable
that fewer ASCs, which reside also around capillaries
and vessels, were isolated from aspirated fat, which
does not contain larger vessels, than excised fat.
Unlike our results, a recent study27 that compared
viable cell yield from fresh aspirated versus fresh
excised fat did not detect a significant difference.
This finding was likely, however, because the study
centrifuged the aspirated fat and removed fibrous
structures and visible vessels from excised fat
during the cell isolation process.
The results of the present study suggested that
addition of ASCs to aspirated fat improved the efficacy
of adipose transfer, though the exact mechanisms
remain to be elucidated. By addition of ASCs to
relatively ASC-poor fat (aspirated fat), the aspirated
fat is theoretically converted to relatively ASC-rich
fat. A recent study using fragmented omentum tissue
suggested the effects of co-transplantation with
preadipocytes, though the fragmented adipose tissue
in that study was not ASC-poor28.
Partly based on the present data, we can speculate
on the fate and roles of ASCs as follows. First
of all, as shown in this study as well as in previous
studies19-21, ASCs can differentiate into vascular
endothelial cells that may contribute to neoangiogenesis
during the healing process after transplantation.
This effect may contribute to the decreased amount
of central necrosis and marked microvasculature
in the outer layers of CAL fat seen in this study.
Second, as suggested in this study, some ASCs were
located between mature adipocytes and in the connective
tissue of CAL fat, as they had been in normal fat
before liposuction. They may play a role as adipose
progenitor cells for future turnover of adipocytes.
The relative deficiency of ASCs in aspirated fat
compared to excised fat may contribute to the low
long-term survival rate in non-CAL fat, which is
a well-known clinical phenomenon25,26. This hypothesis
is also supported by a recent study28 showing that
fragmented omentum (mainly composed of adipose)
with or without preadipocytes (ASCs) was transplanted
and the postoperative atrophy of transplanted tissue
was suppressed when transplanted with preadipocytes.
As it was reported that adipocytes are replaced
with the next generation every 1?2 years in normal
adipose29, the relative deficiency of tissue-specific
progenitor cells in non-CAL fat may affect the coming
turnover of the tissue and lead to its long-term
atrophy. Turnover of adipocytes may occur in the
early stage after transplantation in transplanted
adipose tissue because its vascularity was temporarily
damaged; this outcome may be the reason atrophy
of transplanted adipose is clinically seen during
the first 3 months.
Third, some ASCs may differentiate into mature adipocytes
and partly constitute transplanted fat. Although
adipogenic differentiation of labeled ASCs was not
detected in this study, the failure of detection
may result from difficulty in detecting GFP-labeled
cytoplasm of mature adipocytes, which are filled
with lipid material; indeed, to our knowledge, there
has been no previous report which clearly demonstrated
labeled cytoplasm of mature adipocytes.
Fourth, transplanted ASCs were kept in a hypoxic
condition in the acute phase after transplantation
and may release angiogenic soluble factors such
as VEGF and HGF, accelerating neoangiogenesis from
the surrounding host tissue in a paracrine manner.
It was reported that cultured human ASCs produce
and release VEGF and HGF in a hypoxic condition30.
This effect may also contribute to the decreased
volume of central necrosis and more prominent microvasculature
in the outer layers of the CAL fat seen in this
study.
Surgical injury accompanying the transplantation
and the subsequent hypoxic condition and wound healing
process, including inflammatory reactions, appear
to trigger ASC differentiation into specific lineages
such as adipocytes, vascular endothelial cells,
and mural cells. As ASCs are known to undergo adipogenic
differentiation when co-cultured with mature adipocytes31,
aspirated adipose tissue transplanted together with
ASCs may contribute to acute adipogenic differentiation
of ASCs.
In conclusion, aspirated fat contains less vasculature
and fewer ASCs compared to excised fat. Transplanted
aspirated fat survived better when transplanted
with ASCs than without ASCs. These findings may
partly explain why transplanted aspirated fat does
not survive very well and suggest the clinical potential
of the CAL method. Soft tissue augmentation with
autologous fat, which leaves no incisional scar
and lacks the complications associated with foreign
materials, can be a cosmetically ideal tool when
its effectiveness is improved.
Acknowledgment
GFP rats [SD TgN(act-EGFP)OsbCZ-004] were kindly
provided by Prof. Masaru Okabe (Osaka University,
Japan).
References
1. Zuk, P.A., Zhu, M., Ashjian,
P., De Ugarte, D.A., Huang, J.I., Mizuno, H., Alfonso,
Z.C., Fraser, J.K., Benhaim, P., and Hedrick, M.H.
Human adipose tissue is a source of multipotent
stem cells. Mol. Biol. Cell 13, 4279, 2002.
2. Zuk, P.A., Zhu, M., Mizuno, H., Huang, J., Futrell,
J.W., Katz, A.J., Benhaim, P., Lorenz, H.P., and
Hedrick, M.H. Multilineage cells from human adipose
tissue: implications for cell-based therapies. Tissue
Eng. 7, 211, 2001.
3, Halvorsen, Y.C., Wilkison, W.O., and Gimble,
J.M. Adipose-derived stromal cells--their utility
and potential in bone formation. Int. J. Obes. Relat.
Metab. Disord. 24(Suppl 4), S41, 2004.
4. Dragoo, J.L., Samimi, B., Zhu, M., Hame, S.L.,
Thomas, B.J., Lieberman, J.R., Hedrick, M.H., and
Benhaim, P. Tissue-engineered cartilage and bone
using stem cells from human infrapatellar fat pads.
J. Bone Joint. Surg. Br. 85, 740, 2003.
5. Cowan, C.M., Shi, Y.Y., Aalami, O.O., Chou, Y.F.,
Mar,I, C., Thomas, R., Quarto, N., Contag, C.H.,
Wu, B., and Longaker, M.T. Adipose-derived adult
stromal cells heal critical-size mouse calvarial
defects. Nat. Biotechnol. 22, 560, 2004.
6. Hicok, K.C., Du Laney, T.V., Zhou, Y.S., Halvorsen,
Y.D., Hitt, D.C., Cooper, L.F., and Gimble, J.M.
Human adipose-derived adult stem cells produce osteoid
in vivo. Tissue Eng. 10, 371, 2004.
7. Peterson, B., Zhang, J., Iglesias, R., Kabo,
M., Hedrick, M., Benhaim, P., and Lieberman, J.R.
Healing of critically sized femoral defects, using
genetically modified mesenchymal stem cells from
human adipose tissue. Tissue Eng. 11, 120, 2005.
8. Erickson, G.R., Gimble, J.M., Franklin, D.M.,
Rice, H.E., Awad, H., and Guilak, F. Chondrogenic
potential of adipose tissue-derived stromal cells
in vitro and in vivo. Biochem. Biophys. Res, Commun.
290, 763, 2002.
9. Awad, H.A., Halvorsen, Y.D., Gimble, J.M., and
Guilak, F. Effects of transforming growth factor
beta1 and dexamethasone on the growth and chondrogenic
differentiation of adipose-derived stromal cells.
Tissue Eng. 9, 1301, 2003.
10. Huang, J.I., Zuk, P.A., Jones, N.F., Zhu, M.,
Lorenz, H.P., Hedrick, M.H., and Benhaim, P. Chondrogenic
potential of multipotential cells from human adipose
tissue. Plast. Reconstr. Surg. 113, 585, 2004.
11. Mizuno, H., Zuk, P.A., Zhu, M., Lorenz, H.P.,
Benhaim, P., and Hedrick, M.H. Myogenic differentiation
by human processed lipoaspirate cells. Plast. Reconstr.
Surg. 109, 199, 2002.
12. Rodriguez, A.M., Pisani, D., Dechesne, C.A.,
Turc-Carel, C., Kurzenne, J.Y., Wdziekonski, B.,
Villageois, A., Bagnis, C., Breittmayer, J.P., Groux,
H., Ailhaud, G., and Dani, C. Transplantation of
a multipotent cell population from human adipose
tissue induces dystrophin expression in the immunocompetent
mdx mouse. J. Exp. Med. 201, 1397, 2005.
13. Strem, B.M., Zhu, M., Alfonso, Z., Daniels,
E.J., Schreiber, R., Begyui, R., Maclellan, W.R.,
Hedrick, M.H., and Fraser, J.K. Expression of cardiomyocytic
markers on adipose tissue-derived cells in a murine
model of acute myocardial injury. Cytotherapy 7,
282, 2005.
14. Planat-Benard, V., Menard, C., Andre, M., Puceat,
M., Perez, A., Garcia-Verdugo, J.M., Penicaud, L.,
and Casteilla, L. Spontaneous cardiomyocyte differentiation
from adipose tissue stroma cells. Circ. Res. 94,
223, 2004.
15. Safford, K.M., Hicok, K.C., Safford, S.D., Halvorsen,
Y.D., Wilkison, W.O., Gimble, J.M., and Rice, H.E.
Neurogenic differentiation of murine and human adipose-derived
stromal cells. Biochem. Biophys. Res. Commun. 294,
371, 2002.
16. Ashjian, P.H., Elbarbary, A.S., Edmonds, B.,
De Ugarte, D., Zhu, M., Zuk, P.A., Lorenz, H.P.,
Benhaim, P., and Hedrick, M.H. 2003. In vitro differentiation
of human processed lipoaspirate cells into early
neural progenitors. Plast. Reconstr. Surg. 111,
1922, 2003.
17. Kang, S.K., Lee, D.H., Bae, Y.C., Kim, H.K.,
Baik, S.Y., and Jung, J.S. Improvement of neurological
deficits by intracerebral transplantation of human
adipose tissue-derived stromal cells after cerebral
ischemia in rats. Exp. Neurol. 183, 355, 2003.
18. van Harmelen, V., Skurk, T., and Hauner, H.
Primary culture and differentiation of human adipocyte
precursor cells. Methods Mol. Med. 107, 125, 2005
19. Planat-Benard, V., Silvestre, J.S., Cousin,
B., Andre, M., Nibbelink, M., Tamarat, R., Clergue,
M., Manneville, C., Saillan-Barreau, C., Duriez,
M., Tedgui, A., Levy, B., Penicaud, L., and Casteilla,
L. Plasticity of human adipose lineage cells toward
endothelial cells: physiological and therapeutic
perspectives. Circulation 109, 656, 2004.
20. Miranville, A., Heeschen, C., Sengenes, C.,
Curat, C.A., Busse, R., and Bouloumie, A. Improvement
of postnatal neovascularization by human adipose
tissue-derived stem cells. Circulation 110, 349,
2004.
21. Cao Y, Sun Z, Liao L, Meng Y, Han Q, Zhao RC.
2005. Human adipose tissue-derived stem cells differentiate
into endothelial cells in vitro and improve postnatal
neovascularization in vivo. Biochem Biophys Res
Commun. 332:370-9.
22. Yoshimura, K., Shigeura, T., Matsumoto, D.,
Sato, T., Takaki, Y., Aiba-Kojima, E., Sato, K.,
Inoue, K., Nagase, T., Koshima, I, and Gonda, K.
Characterization of Freshly Isolated and Cultured
Cells Derived from the Fatty and Fluid Portions
of Liposuction Aspirates. J. Cell. Physiol., in
press.
23. Lendeckel, S., Jodicke, A., Christophis, P.,
Heidinger, K., Wolff, J., Fraser, J.K., Hedrick,
M.H., Berthold, L., and Howaldt, H.P. Autologous
stem cells (adipose) and fibrin glue used to treat
widespread traumatic calvarial defects: case report.
J. Craniomaxillofac. Surg. 32, 370, 2004.
24. Garcia-Olmo, D., Garcia-Arranz, M., Herreros,
D., Pascual, I., Peiro, C., Rodriguez-Montes, J.A.
A phase I clinical trial of the treatment of Crohn's
fistula by adipose mesenchymal stem cell transplantation.
Dis. Colon Rectum 48, 1416, 2005.
25. Ersek, R.A., Chang, P., and Salisbury, M.A.
Lipo layering of autologous fat: an improved technique
with promising results. Plast. Reconstr. Surg. 101,
820, 1998.
26. Shiffman, M.A., and Mirrafati, S. Fat transfer
techniques: the effect of harvest and transfer methods
on adipocyte viability and review of the literature.
Dermatol. Surg. 27, 819, 2001.
27. von Heimburg, D., Hemmrich, K., Haydarlioglu,
S., Staiger, H., and Pallua, N. Comparison of viable
cell yield from excised versus aspirated adipose
tissue.
Cells Tissues Organs 178, 87, 2004.
28. Masuda, T., Furue, M., Matsuda, T. Novel strategy
for soft tissue augmentation based on transplantation
of fragmented omentum and preadipocytes. Tissue
Eng. 10, 1672, 2004.
29. Strawford, A., Antelo, F., Christiansen, M.,
and Hellerstein, M.K. Adipose tissue triglyceride
turnover, de novo lipogenesis, and cell proliferation
in humans measured with 2H2O. Am. J. Physiol. Endocrinol.
Metab. 286, E577, 2004.
30. Rehman, J., Traktuev, D., Li, J., Merfeld-Clauss,
S., Temm-Grove, C.J., Bovenkerk, J.E., Pell, C.L,,
Johnstone, B.H., Considine, R.V., and March, K.L.
Secretion of angiogenic and antiapoptotic factors
by human adipose stromal cells. Circulation 109,
1292, 2004.
31. Considine, R.V., Nyce, M.R., Morales, L.M.,
Magosin, S.A., Sinha, M.K., Bauer, T.L., Rosato,
E.L., Colberg, J., and Caro, J.F. Paracrine stimulation
of preadipocyte-enriched cell cultures by mature
adipocytes. Am. J. Physiol. 270, E895, 1996.
Figure Legends
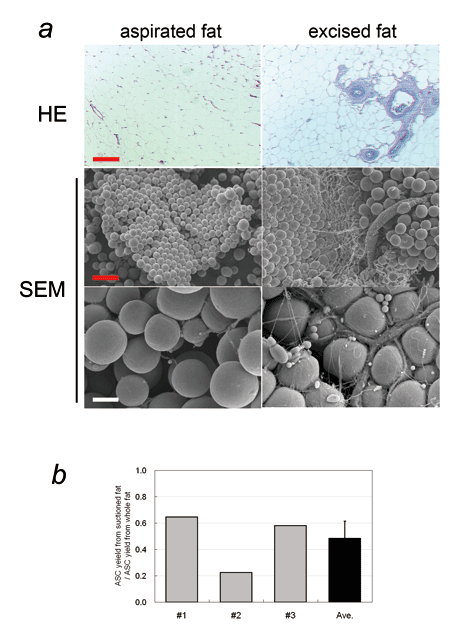
Fig. 1. Comparison of human aspirated fat and excised
whole fat obtained from a single site of a single
patient.
(a) Histology of aspirated fat and excised fat.
(HE stained microphotographs and SEM photos; red
scale bar = 200 Κm, white scale bar = 40 Κm). The
basic structure of adipose tissue was preserved
in the aspirated fat, while vascular vessels, especially
those of large size, were significantly less detected
in aspirated fat compared to the excised fat. It
is well known that the honeycomb structures of vascular
and neural perforator networks are left intact in
aspirated sites after liposuction operation (Fig.
S1).
(b) ASC yield from aspirated fat and excised fat.
Both tissues were processed for isolation of SVFs,
which were then cultured for 1 week. Ratios of ASC
yield from aspirated fat to that from excised fat
of the same volume were calculated; data from three
patients (#1-#3) and their average value were demonstrated.
ASC yield from aspirated fat was significantly less
(48 } 13 %) than that from excised fat.
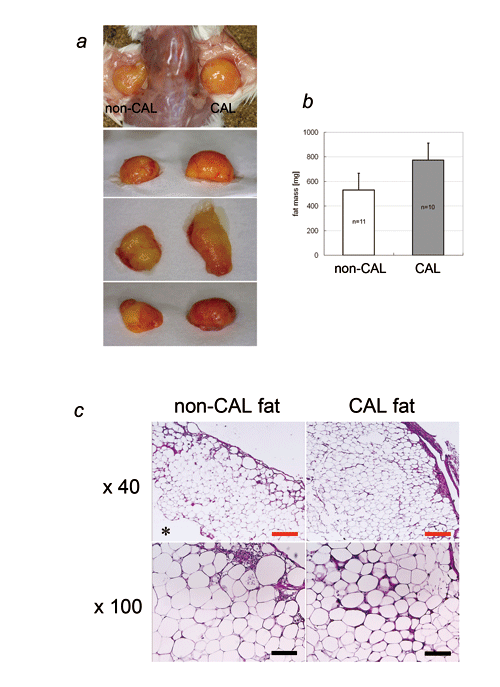
Fig. 2. CAL and non-CAL using human aspirated fat
and SVF.
(a) Photographs of CAL fat (right side) and non-CAL
fat (left side) in SCID mice. Human aspirated fat
was transplanted with (CAL fat) or without (non-CAL
fat) human SVFs freshly isolated from the same aspirated
fat. Transplanted fat was harvested at 4 weeks.
(b) Weight of harvested CAL fat and non-CAL fat.
The weight of CAL fat was significantly greater
than that of non-CAL fat (p < 0.05).
(c) Histology of CAL fat and non-CAL fat samples
(red scale bar = 250 Κm, black scale bar = 100 Κm).
Central necrosis (*) was almost always seen in non-CAL
fat, and the surviving layer of CAL fat was thicker
than that of non-CAL fat. At higher magnification,
microvasculature was more frequently seen in CAL
fat, especially in the outer layers, than in non-CAL
fat.
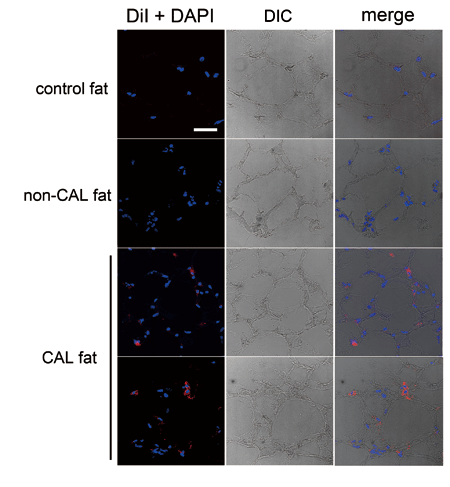
Fig. 3. Histology of CAL fat using human aspirated
fat and DiI-labeled SVF. Normal excised fat and
non-CAL fat were also used for comparison. Nuclei
were stained with DAPI. In CAL fat, DiI-labeled
cells, which are supposed to be co-transplanted
ASCs, are located between mature adipocytes and
in interstitial connective tissue. The frequency
of DiI-positive cells was 10%?30%, though it varied
among samples and at locations in a sample. DIC:
differential interference contrast. Scale bar =
50 Κm. The right column shows merges of DiI and
DAPI, and DIC images.
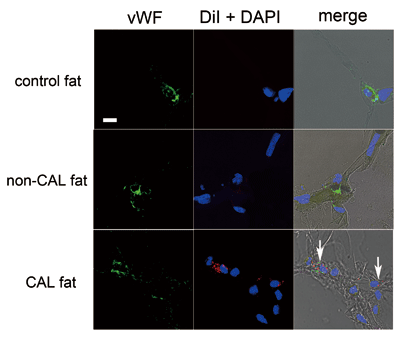
Fig. 4. Immunohistology of CAL fat using human aspirated
fat and DiI-labeled SVF. Normal excised fat (control
fat) and non-CAL fat were also assessed for comparison.
Endothelial cells were immunostained with vWF. Nuclei
were stained with DAPI. Cells double-positive for
vWF and DiI (arrows), which were suggested to be
vascular endothelial cells differentiated from co-transplanted
DiI-labeled ASCs, were observed in connective tissue
or between adipocytes. Scale bar = 10 Κm. The right
column shows merges of immunostaining of vWF, DiI,
DAPI, and DIC images.
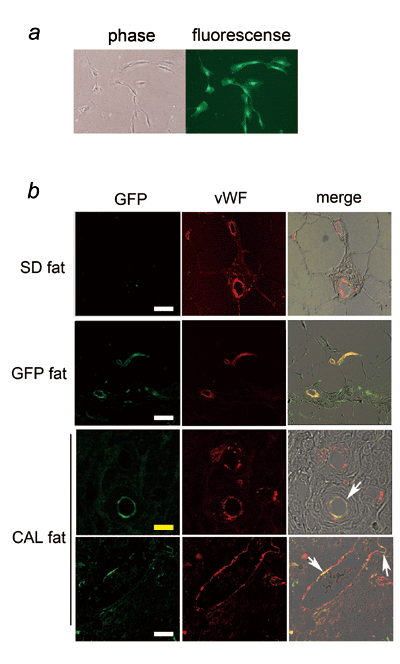
Fig. 5. CAL fat in rat models (fragmented fat of
SD rat and GFP-SVF).
(a) Cultured GFP-ASCs (phase-contrast and fluorescence
images). When GFP-SVF cells were cultured, all adherent
cells were GFP-positive.
(b) Immunohistology of CAL fat in rat models. GFP
was detected by immunostaining with anti-GFP antibody.
Adipose tissue of SD rat and GFP rat were also demonstrated
for comparison. Vascular endothelial cells are only
vWF-positive in SD fat, while they were all double
positive for vWF and GFP in GFP fat. In CAL fat,
capillaries with endothelial cells partly or entirely
double positive for GFP and vWF (arrows) were detected,
suggesting that they were differentiated from co-transplanted
GFP-ASCs. The other endothelial cells were only
vWF-positive, suggesting that they were derived
from the host SD rat. White scale bar = 30 Κm, yellow
scale bar = 10 Κm. The right column shows merges
of immunostaining of vWF, DiI, DAPI, and DIC images.
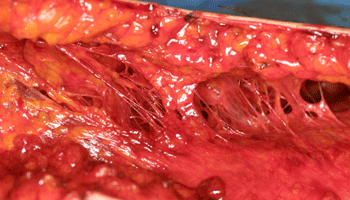
Fig. S1. Honeycomb structures in subcutaneous layers
after liposuction. Vascular and neural perforators
arising from the fascia or muscle are left intact
after suctioning. Liposuction is usually performed
with a metal cannula after infiltration of saline
solution containing lidocaine and adrenaline. This
fact clearly supports that aspirated fat contains
fewer vascular structures compared to excised fat.