Rapid
expansion of human adipose-derived stromal cells
preserving multipotency
Hirotaka Suga, Tomokuni
Shigeura,b Daisuke Matsumoto,a Keita Inoue, Harunosuke
Kato, Noriyuki Aoi, Shoko Murase, Katsujiro Sato,
Koichi Gonda, Isao Koshima, and Kotaro Yoshimura |
Introduction
Human adherent stromal cells isolated from adipose tissue
have been shown to have multipotency [1, 2], able to differentiate
not only into mesenchymal lineages including endothelial
cells [3-6] and cardiomyocytes [7-9] but also into neural
cells [2] and hepatocytes [10]. These cells have been referred
to by various names, including preadipocytes, vascular stromal
cells, adipose-derived mesenchymal progenitor cells, and
adipose stromal cells. In this study, we refer to the cells
as adipose-derived stromal (stem) cells (ASCs). The characteristics
of ASCs have been extensively studied [11-15], as well as
the potential clinical applications of ASCs [3-7, 16]. In
addition, clinical trials have already begun involving enhancement
of bone and adipose regeneration and angiogenesis [17-20].
Adipose tissue is thought to be a promising source of multipotent
stromal cells because it can be harvested in relatively
large quantities (100 mL to > 1 L) using liposuction
with minimal morbidity. Although ASCs may be clinically
used without cell expansion because of their large quantities,
it is of great value to culture and expand ASCs safely and
effectively without losing their multipotency for manipulation
and further development of cell-based therapies. There have
been some reports indicating enhanced proliferation of human
ASCs using specific culture media with supplements. It was
shown that fibroblast growth factor (FGF)-2 was released
by ASCs, enhanced proliferation [21-24], and maintained
the adipogenic potential of ASCs [21]. FGF-1 and epidermal
growth factor (EGF) were suggested to act as stimulators
of both ASC proliferation and differentiation [25-27]. Platelet
-derived growth factor (PDGF) [25, 28], tumor necrosis factor
(TNF)-α [29], and insulin-like growth factor (IGF)-1 [29]
were also shown to promote ASC proliferation, and the former
two factors were suggested to have inhibiting effects on
ASC differentiation [25, 29]. However, it has not been shown
whether ASCs expanded by these methods preserve their multipotency
or not.
In this study, we investigated the effects of an endothelial
growth medium (EGM-2R, Cambrex, Walkersville, MD) on culturing
human ASCs, focusing on proliferation and differentiation
potentials, and found that it markedly expands ASCs preserving
multipotency. EGM-2 is usually used to support the growth
of endothelial cells. In recent studies, EGM-2 has been
used for culture of non-endothelial cells [16, 30, 31].
However, ASCs have usually been cultured in Dulbecco’s modified
Eagle’s medium (DMEM) or DMEM/F12 medium, and the effects
of EGM-2 on ASCs have not been reported.
Materials and methods
Cell isolation and culture
We obtained liposuction aspirates from 12 healthy female
donors undergoing liposuction of the abdomen or thighs after
informed consent using an IRB-approved protocol. The stromal
vascular fraction containing ASCs was isolated from the
fatty portion of liposuction aspirates, as previously described
[15]. Briefly, the aspirated fat was washed with phosphate
buffered saline (PBS) and digested on a shaker at 37oC in
PBS containing 0.075% collagenase for 30 min. Mature adipocytes
and connective tissues were separated from pellets by centrifugation
(800×g, 10 min). The cell pellets were resuspended, filtered
with a 100-μm mesh (Millipore, MA, USA), plated at a density
of 5×105 nucleated cells/100-mm dish, and cultured at 37°C
in an atmosphere of 5% CO2 in humid air. The culture medium
was: (1) DMEM (Nissui Pharmaceutical, Tokyo, Japan) containing
10% fetal bovine serum (FBS), or (2) EGM-2 containing 2%
FBS. Endothelial basal medium (EBM, Cambrex) is a basal
medium for EGM-2. EGM-2 does not contain any animal-derived
factors but does contain FGF-2, vascular endothelial growth
factor (VEGF), IGF-1, EGF, ascorbic acid, hydrocortisone,
GA-1000 (gentamicin and amphotericin-B), and heparin, although
the concentration of each agent is not disclosed. Freshly
isolated cells were cultured for 7 days and the first culture
was defined as “Passage 0.” The medium was replaced every
3 days. Cells were passaged every week by trypsinization.
Measurement of doubling time
and total cell number
During cell culture in each medium, doubling time was measured
at passages 0, 1, 2, and 3 by seeding ASCs at a density
of 1×105 cells per 10-cm dish. After cells reached the logarithmic
growth phase, they were sequentially trypsinized every 48
h and counted with a cell counter (NucleoCounter, Chemometec,
Allerod, Denmark). Doubling time was calculated according
to the following formula: doubling time = 48 h/log2(N2/N1),
where N1 is the first cell count and N2 is the cell count
48 h later. Total cell number after the initiation of culture
in each medium was also measured by seeding ASCs (Passage
0) at a density of 1×104 cells per 3.5-cm dish and culturing
the cells until they reached the stationary phase.
Measurement of the proliferative
effect of supplemented growth factors
To examine the proliferative effect of each growth factor
supplemented in EGM-2, ASCs were cultured in medium supplemented
with a single growth factor (VEGF, EGF, IGF-1, or FGF-2).
EBM containing 2% FBS was used as the control medium. ASCs
(Passage 0) were seeded at a density of 1×104 cells per
well in a 6-well plate. Cells were cultured in the control
medium (2% FBS), supplemented medium (0.1, 1, or 10 ng/ml
of each growth factor) (2% FBS), DMEM (10% FBS), or EGM-2
(2% FBS). The number of cells after 7 days of culture was
counted using a cell counter.
Flow cytometry of cultured
cells
Cultured cells in each medium were examined for surface
marker expression using flow cytometry. The following monoclonal
antibodies (MAbs) conjugated to fluorochromes were used:
anti-CD29-PE, CD31-PE, CD34-PE, CD45-PE, CD90-PE, CD146-PE
(BD Biosciences, San Diego, CA), CD105-PE (Serotec, Oxford,
UK), and Flk-1-PE (Techne, Minneapolis, MN). Control MAbs
were included for all fluorochromes. Cells were incubated
with directly conjugated MAbs for 30 minutes, then washed
and fixed in 1% paraformaldehyde. Cells were analyzed using
an LSR II (Becton Dickinson, San Jose, CA) flow cytometry
system. Data acquisition and analysis were then performed
(Cell Quest software, Becton Dickinson). Gates were set
based on staining with combinations of relevant and irrelevant
MAbs so that no more than 0.1% of cells were positive using
irrelevant antibodies.
Induced differentiation of
cultured cells
After culture in each medium for 2 weeks, differentiation
into the adipogenic, chondrogenic, and osteogenic lineages
was examined.
For adipogenic differentiation, cells were incubated for
4 weeks in DMEM containing 10% FBS supplemented with 0.5
mM isobutyl-methylxanthine (Sigma, St. Louis, MO), 1 ?M
dexamethasone, 10 ?M insulin (Sigma), and 200 ?M indomethacin.
Adipogenic differentiation was visualized with oil red O
staining. For quantitative analysis of lipid droplets, we
measured Nile Red fluorescence, using AdipoRedTM (Cambrex),
with excitation at 485 nm and emission at 535 nm.
For chondrogenic differentiation, cells were incubated for
4 weeks in DMEM containing 1% FBS supplemented with 6.25
?g/ml insulin, 10 ng/ml TGF-β1, and 50 nM ascorbate-2-phosphate.
Chondrogenic differentiation was visualized with Alcian
Blue staining. For quantitative analysis, a micromass culture
system was used as previously reported [32]. Cells were
plated in a 15-ml tube and cultured in the chondrogenic
medium for 3 weeks. Then, the diameter of a micromass was
measured.
For osteogenic differentiation, cells were incubated for
4 weeks in DMEM containing 10% FBS supplemented with 0.1
μM dexamethasone, 50 μM ascorbate-2-phosphate, and 10 mM
β-glycerophosphate (Nacalai Tesque, Kyoto, Japan). Osteogenic
differentiation was visualized with von Kossa staining.
For quantitative analysis of total calcium, calcium deposition
was evaluated based on the ortho-cresolphthalein complexone
(OCPC) method with the Calcium C-Test Wako Kit (Wako Chemicals)
according to the manufacturer’s instructions.
Statistical analyses
Results were expressed as mean ± SEM. Welch’s t-test was
used to compare each parameter. A value of p < 0.05 was
considered significant.
Results
Doubling time and total cell number
Doubling time of ASCs cultured with EGM-2 was significantly
shorter than that of cells cultured with DMEM at each passage
(19.3 ± 2.1 h vs. 39.8 ± 6.8 h at Passage 0; 15.6 ± 1.1
h vs. 55.1 ± 3.5 h at Passage 1; 20.3 ± 0.7 h vs. 52.0 ±
2.4 h at Passage 2; and 26.5 ± 1.1 h vs. 54.3 ± 6.4 h at
Passage 3) (Fig. 1A). Total cell number showed that ASCs
cultured with EGM-2 proliferated more rapidly and reached
the stationary phase earlier than those cultured with DMEM
(40 days vs. 200 days), though the maximum population doubling
level of ASCs was similar either when cultured with EGM-2
or DMEM (35?40 with EGM-2 vs. 40?45 with DMEM) (Fig. 1B).
Differentiation assays were performed using ASCs cultured
with each medium for 2 weeks, and at that stage, ASCs cultured
with EGM-2 were supposed to be expanded 105 times (1010
vs. 105) compared to those cultured with DMEM (Fig. 1B).
Proliferative effect of each
growth factor
At the concentrations tested, VEGF, EGF, and IGF-1 showed
no significant proliferative effect on ASCs cultured in
EBM containing 2% FBS. FGF-2 at a density of 0.1, 1, or
10 ng/ml significantly promoted proliferation of ASCs compared
to control. However, the proliferative effect of FGF-2 was
much less than that of EGM-2 containing all of the growth
factors, indicating a synergistic effect of supplemented
growth factors (Fig. 2).
Flow cytometry
Flow cytometry of ASCs cultured in DMEM and EGM-2 showed
no significant differences except CD 105 at passages 1,
2, and 3 (Table 1). Both cell populations uniformly expressed
mesenchymal markers (CD29 and CD90) and were devoid of a
hematopoietic cell marker CD45. Expressions of CD34 (stem
cell marker), CD31 (endothelial cell marker), CD146 (endothelial
cell and vascular mural cell marker), and Flk-1 (VEGFR-2)
were similar in both cell populations, and CD34 expression
of ASCs markedly decreased at passage 1 in both media.
Differentiation capacity
Both cell populations cultured in DMEM and EGM-2 for 2 weeks
had similar capacities to differentiate into adipogenic,
chondrogenic, and osteogenic lineages. No morphological
differences between the two cell populations were observed
during and after differentiation (Fig. 3A). Quantitative
analyses (lipid droplets in adipogenic differentiation,
micromass diameter in chondrogenic differentiation, and
total calcium content in osteogenic differentiation) also
showed no significant differences between the two cell populations
(Fig. 3B).
Discussion
In this study, EGM-2 expanded ASCs very rapidly while preserving
their multipotency for at least 2 weeks; the proliferative
efficiency of EGM-2 was 105 times of that of DMEM in the
first 2 weeks. A doubling time of ASCs shorter than that
shown in this study (15?20 hours) has not been reported
previously in the literature. EGM-2 contains 2% FBS and
various growth factors including FGF-2, VEGF, IGF-1, and
EGF. The highly boosting effects of EGM-2 on ASC proliferation
are suggested to result from supplemented growth factors
and other unknown synergistic effects, as discussed below.
ASCs cultured with EGM-2 proliferated much more rapidly
and reached the stationary phase earlier than those cultured
with DMEM (40 days vs. 200 days), although the maximum population
doubling levels were similar between the two culture media
(Fig. 1B). The results suggest that a majority of ASCs may
have a limited capacity of self renewal.
Serum concentrations can affect proliferation activity of
ASCs. We previously reported that the doubling time of ASCs
cultured with 15% FBS was significantly shorter than that
of cells cultured with 10% FBS, although the culture media
was M199 supplemented with FGF-1 in that study [15]. FBS
is made through coagulations of fetal bovine whole blood;
thus, it is supposed to contain not only IGF-1, which is
regularly present in serum including platelet-poor plasma-derived
serum, but also platelet-derived cytokines such as PDGF
and EGF (our unpublished data, in submission). In the present
study, however, the doubling time of ASCs cultured in DMEM
was significantly longer than that for ASCs cultured in
EGM-2, in spite of the higher FBS concentration (10%) in
DMEM compared to EGM-2 (2%). This result suggests that the
growth factors added to EGM-2 have greater effects on the
proliferation activity of ASCs than do serum concentrations.
In fact, a recent report suggested that platelet-derived
growth factors (not designated but assumed to be PDGF and
EGF) may reduce proliferation activity and adipogenic differentiation
capacity of ASCs [23].
Among growth factors contained in EGM-2, supplementation
with IGF-1, EGF, or VEGF (0.1, 1, or 10 ng/ml of each growth
factor) did not significantly promote proliferation activity
of ASCs in EBM culture containing 2% FBS. The growth factors
have some proliferative effects on ASCs when added to serum-free
media or SPPP, as reported previously [5, 23, 29]; however,
any effects in this study using a low concentration of FBS
with IGF-1 and EGF were subtle or masked. In this study,
only FGF-2 showed a statistically significant promoting
effect on ASC proliferation, which has been suggested in
previous studies [21-24]. The results strongly suggest that
FGF-2 is a critical growth factor for supplementation of
serum-containing culture media. A previous study suggested
that FGF-2 plays a critical role in self renewal of ASCs
[21]. It was also shown that FGF-2 added to SPPP increased
proliferation activity and adipogenic differentiation capacity
[23]. Another study reported efficient proliferation of
ASCs transfected with the FGF-2 gene [33]. However, in 7
days, EGM-2 expanded ASCs significantly and several-fold
more compared to FGF-2-supplemented EBM (Fig. 2), and thus
the effect of EGM-2 on ASC proliferation cannot be explained
solely based on the influence of FGF-2 alone. It is likely
that synergistic effects of various growth factors and other
factors contributed to the exceptional efficiency.
We previously reported surface marker expression of freshly
isolated and cultured ASCs [15], although M-199 was used
in the study. In the present study, the character of ASCs
expanded with EGM-2 did not appear to change significantly.
ASCs cultured with EGM-2 preserved differentiation capacities
similar to those with DMEM at least into three mesenchymal
lineages: adipogenic, chondrogenic, and osteogenic. In addition,
flow cytometry of both populations showed no significant
differences except for CD105 and presented no increase of
differentiation markers such as CD31, suggesting that ASCs
remain in an undifferentiated and proliferating state. These
results suggest that EGM-2 accelerates expansion of ASCs
mainly by facilitating proliferation of undifferentiated
cells. Although expression of CD105 has been used as one
of definitive markers of mesenchymal stem cells, it may
not strongly correlate with the multipotency.
Recent reports have shown that ASCs can differentiate into
endothelial cells in vitro under certain culture conditions
using endothelial growth media and also in vivo [3-6]. ASCs
may be essentially common progenitors of adipocytes and
vascular cells [5]. In most of the in vitro studies, a semisolid
medium like methylcellulose or Matrigel was used, which
may be key to endothelial differentiation of ASCs [34].
Although EGM-2 containing VEGF was originally a medium for
expanding endothelial cells and ASCs express Flk-1, a VEGF
receptor, endothelial cell marker expression of ASCs was
not enhanced by EGM-2 in our study using cell culture on
a plastic dish. In addition, hypoxic conditions have various
influences on ASCs [35-38], one of which is enhancing ASC
secretion of angiogenic factors such as VEGF and HGF [35].
In the studies showing endothelial differentiation of ASCs
in vivo, ASCs were transplanted to the ischemic hindlimb
or under other ischemic conditions [4-6], so that a hypoxic
condition may be an important factor in endothelial differentiation
in vivo.
A number of preclinical studies with human ASCs have been
reported; in most, undifferentiated ASCs were used, rather
than ASCs differentiated into a specific lineage, although
the functional mechanism of transplanted ASCs varied among
studies. Transplanted ASCs survive as undifferentiated cells
and act as tissue-specific progenitors or provider cells
of soluble factors in some studies [3, 7, 16]. In others,
transplanted ASCs differentiated into a specific lineage
such as bone and vessels according to the circumstances
of recipient sites [3, 7, 17]. In the therapeutic use of
ASCs, expansion of undifferentiated cells, rather than their
differentiation into a specific lineage, is likely of great
importance in the processing of the cells before transplantation.
In clinical practice, a safer and more rapid expansion method
is required in view of time and cost requirements. Although
both EBM and EGM-2 are not approved for clinical use at
present, EGM-2 does not contain animal-derived factors,
and the FBS used in this study can be easily replaced with
autologous serum or human allogenic serum. The present expansion
method with EGM-2 has an exceptional efficiency and lays
the groundwork for establishing a practical route to mega-expansion
of ASCs for clinical applications.
Acknowledgments
We are very grateful to Ayako Kurata, Akiko Matsuura, and
Satomi Kawarasaki for their technical assistance.
References
1. Zuk PA, Zhu M, Mizuno H, et al. Multilineage cells from
human adipose tissue: implications for cell-based therapies.
Tissue Eng 2001;7:211-228.
2. Zuk PA, Zhu M, Ashjian P, et al. Human adipose tissue
is a source of multipotent stem cells. Mol Biol Cell 2002;13:4279-4295.
3. Matsumoto D, Sato K, Gonda K, et al. Cell-assisted lipotransfer:
supportive use of human adipose-derived cells for soft tissue
augmentation with lipoinjection. Tissue Eng 2006;12:3375-3382.
4. Planat-Benard V, Silvestre JS, Cousin B, et al. Plasticity
of human adipose lineage cells toward endothelial cells:
physiological and therapeutic perspectives. Circulation
2004;109:656-663.
5. Miranville A, Heeschen C, Sengenes C, et al. Improvement
of postnatal neovascularization by human adipose tissue-derived
stem cells. Circulation 2004;110:349-355.
6. Cao Y, Sun Z, Liao L, et al. Human adipose tissue-derived
stem cells differentiate into endothelial cells in vitro
and improve postnatal neovascularization in vivo. Biochem
Biophys Res Commun 2005;332:370-379.
7. Miyahara Y, Nagaya N, Kataoka M, et al. Monolayered mesenchymal
stem cells repair scarred myocardium after myocardial infarction.
Nat Med 2006;12:459-465.
8. Fraser JK, Schreiber R, Strem B, et al. Plasticity of
human adipose stem cells toward endothelial cells and cardiomyocytes.
Nat Clin Pract Cardiovasc Med 2006;3:S33-S37.
9. Planat-Benard V, Menard C, Andre M, et al. Spontaneous
cardiomyocyte differentiation from adipose tissue stroma
cells. Circ Res 2004;94:223-229.
10. Seo MJ, Suh SY, Bae YC, et al. Differentiation of human
adipose stromal cells into hepatic lineage in vitro and
in vivo. Biochem Biophys Res Commun 2005;328:258-264.
11. Gronthos S, Franklin DM, Leddy HA, et al. Surface protein
characterization of human adipose tissue-derived stromal
cells. J Cell Physiol 2001;189:54-63.
12. Katz AJ, Tholpady A, Tholpady SS, et al. Cell surface
and transcriptional characterization of human adipose-derived
adherent stromal (hADAS) cells. Stem Cells 2005;23:412-423.
13. Tholpady SS, Llull R, Ogle RC, et al. Adipose tissue:
stem cells and beyond. Clin Plast Surg 2006;33:55-62.
14. Sengenes C, Lolmede K, Zakaroff-Girard A, et al. Preadipocytes
in the human subcutaneous adipose tissue display distinct
features from the adult mesenchymal and hematopoietic stem
cells. J Cell Physiol 2005;205:114-122.
15. Yoshimura K, Shigeura T, Matsumoto D, et al. Characterization
of freshly isolated and cultured cells derived from the
fatty and fluid portions of liposuction aspirates. J Cell
Physiol 2006;208:64-76.
16. Nakagami H, Maeda K, Morishita R, et al. Novel autologous
cell therapy in ischemic limb disease through growth factor
secretion by cultured adipose tissue-derived stromal cells.
Arterioscler Thromb Vasc Biol 2005;25:2542-2547.
17. Lendeckel S, Jodicke A, Christophis P, et al. Autologous
stem cells (adipose) and fibrin glue used to treat widespread
traumatic calvarial defects: case report. J Craniomaxillofac
Surg 2004;32:370-373.
18. Garcia-Olmo D, Garcia-Arranz M, Herreros D, et al. A
phase I clinical trial of the treatment of Crohn’s fistula
by adipose mesenchymal stem cell transplantation. Dis Colon
Rectum 2005;48:1416-1423.
19. Yoshimura K, Matsumoto D, Gonda K. A clinical trial
of soft tissue augmentation by lipoinjection with adipose-derived
stromal cells (ASCs). Proceedings of the 8th annual meeting
of Tissue Engineering Society International (TESI), 2005;
pp206-207, Shanhai, China.
20. Moseley TA, Zhu M, Hedrick MH. Adipose-derived stem
and progenitor cells as fillers in plastic and reconstructive
surgery. Plast Reconstr Surg 2006;118:S121-S128.
21. Zaragosi L, Ailhaud G, Dani C. Autocrine fibroblast
growth factor 2 signaling is critical for self-renewal of
human multipotent adipose-derived stem cells. Stem Cells
2006;24:2412-2419.
22. Quarto N, Longaker MT. FGF-2 inhibits osteogenesis in
mouse adipose tissue-derived stromal cells and sustains
their proliferative and osteogenic potential state. Tissue
Eng 2006;12:1-14.
23. Koellensperger E, Heimburg DV, Markowicz M, et al. Human
serum from platelet-poor plasma for the culture of primary
human preadipocytes. Stem Cells 2006;24:1218-1225.
24. Chiou M, Xu Y, Longaker MT. Mitogenic and chondrogenic
effects of fibroblast growth factor-2 in adipose-derived
mesenchymal cells. Biochem Biophys Res Commun 2006;343:644-652.
25. Hauner H, Rohrig K, Petruschke T. Effects of epidermal
growth factor (EGF), platelet-derived growth factor (PDGF)
and fibroblast growth factor (FGF) on human adipocyte development
and function. Eur J Clin Invest 1995;25:90-96.
26. Hutley L, Shurety W, Newell F, et al. Fibroblast growth
factor 1: a key regulator of human adipogenesis. Diabetes
2004;53:3097-3106.
27. Serrero G.. EGF inhibits the differentiation of adipocyte
precursors in primary cultures. Biochem Biophys Res Commun
1987;146:194-202.
28. Kang YJ, Jeon ES, Song HY, et al. Role of c-Jun N-terminal
kinase in the PDGF-induced proliferation and migration of
human adipose tissue-derived mesenchymal stem cells. J Cell
Biochem 2005;95:1135-1145.
29. Kras KM, Hausman DB, Martin RJ. Tumor necrosis factor-?
stimulates cell proliferation in adipose tissue-derived
stromal-vascular cell culture: promotion of adipose tissue
expansion by paracrine growth factors. Obes Res 2000;8:186-193.
30. Simper D, Stalboerger PG, Panetta CT, et al. Smooth
muscle progenitor cells in human blood. Circulation 2002;106:1199-1204.
31. Zhang R, Yang H, Li M, et al. Acceleration of endothelial-like
cell differentiation from CD14+ monocytes in vitro. Exp
Hematol 2005;33:1554-1563.
32. Johnstome B, Hering TM, Caplan AI, et al. In vitro chondrohenesis
of bone marrow-derived mesenchymal progenitor cells. Exp
Cell Res 1998;238:265-272.
33. Yamashiro H, Inamoto T, Yagi M, et al. Efficient proliferation
and adipose defferentiation of human adipose tissue-derived
vascular stromal cells transfected with basic fibroblast
growth factor gene. Tissue Eng 2003;9:881-892.
34. Gehling UM, Ergun S, Schumacher U, et al. In vitro differentiation
of endothelial cells from AC133-positive progenitor cells.
Blood 2000;95:3106-3112.
35. Rehman J, Traktuev D, Li J, et al. Secretion of angiogenic
and antiapoptotic factors by human adipose stromal cells.
Circulation 2004;109:1292-1298.
36. Kim KH, Song MJ, Chung J, et al. Hypoxia inhibits adipocyte
differentiation in a HDAC-independent manner. Biochem Biophys
Res Commun 2005;333:1178-1184.
37. Wang DW, Fermor B, Gimble JM, et al. Influence of oxygen
on the proliferation and metabolism of adipose derived adult
stem cells. J Cell Physiol 2005;204:184-191.
38. Lee JH, Kemp DM. Human adipose-derived stem cells display
myogenic potential and perturbed function in hypoxic conditions.
Biochem Biophys Res Commun 2006;341:882-888.
Figure legends
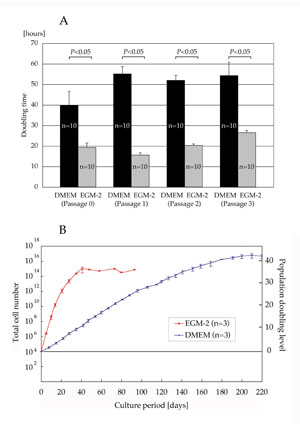
Fig. 1. (A) Doubling time at passages 0?3. Doubling time
of ASCs cultured with EGM-2 was significantly shorter than
for those cultured with DMEM at each passage.
(B) Total cell number and population doubling level after
the initiation of culture with DMEM or EGM-2. ASCs cultured
in EGM-2 proliferated more rapidly and reached the stationary
phase earlier than those cultured in DMEM. After 40 days
of culture with EGM-2, the result was obtained from one
sample.
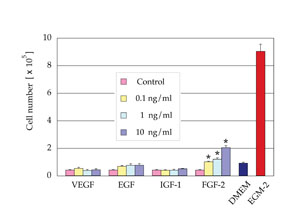
Fig. 2. Cell number after 7 days of culture in EBM (2% FBS)
supplemented with one of the following growth factors, VEGF,
EGF, IGF-1, or FGF-2 (n=3). FGF-2 at a density of 0.1, 1,
or 10 ng/ml significantly promoted proliferation of ASCs
compared to control medium. The numbers of cells cultured
in DMEM (10% FBS) or EGM-2 (2% FBS) are also indicated.
*: p < 0.05.
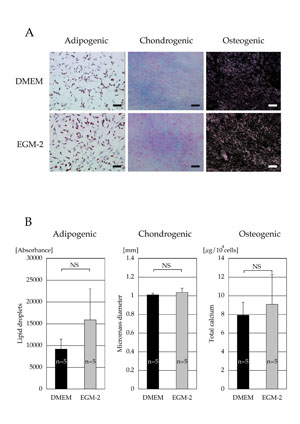
Fig. 3. (A) Microscopic results of cell differentiation.
Both cell populations cultured in DMEM and EGM-2 for 2 weeks
had similar capacities to differentiate into adipogenic,
chondrogenic, and osteogenic lineages. Adipogenic, chondrogenic,
and osteogenic differentiations were visualized with oil
red O staining, Alcian Blue staining, and von Kossa staining,
respectively. Scale bar = 100 μm.
(B) Quantitative analyses of cell differentiation. Differentiation
potentials were evaluated by lipid droplet contents (adipogenic),
micromass diameter (chondrogenic), and total calcium contents
(osteogenic). No statistical significances were observed
(adipogenic, p = 0.31: chondrogenic, p = 0.68, and osteogenic,
p = 0.55). NS: no significant difference.
Table 1.
Flow cytometry analyses of cell surface marker antigens.
Expression of mesenchymal markers (CD29, CD90, CD105), endothelial
markers (CD31, CD146, Flk-1), a stem cell marker (CD34),
and a hematopoietic marker (CD45) of ASCs cultured with
DMEM or EGM-2 was quantitatively examined at passages 0?3.
|