Introduction
One of the requirements for successful cell-based
therapy is the delivery of stem cells to target tissues
after manipulations such as the expansion stem cell
cultures or commitment of stem cells to a specific
lineage. However, due to safety considerations such
as transmission of viral or prion-related disease,
the use of animal-derived serum, tissue extracts,
enzymes and cytokines in these manipulations is undesirable,
so autologous, human-derived substitutes for these
animal products could be very useful. Several studies
have examined the use of alternatives: Attempts were
made to use human autoserum as a replacement for fetal
bovine serum (FBS) (McAlinden et al., 2000), though
its volume available is limited. Patient-derived fibrin
glue (thrombin and fibrinogen) and platelet-rich plasma
have also been used for cell culture or in clinical
trials for enhancing tissue regeneration (Thor et
al., 2005). Other studies have shown that growth factors
derived from platelets can be used to stimulate cell
proliferation (Ross et al., 1974; Lucarelli et al.,
2003; Eppley et al., 2004), but platelets can not
provide some other major growth factors such as b-FGF,
KGF, and HGF (Sanchez et al, 2003). In this study,
we examined the biochemical profiles of wound drainage
fluids and compared them to those of serum derived
from platelet-rich and platelet-poor plasma in order
to assess whether these drainage fluids could be used
as a supplement and/or replacement for animal-derived
factors in situations in which cell culture would
ideally be carried out in the absence of such animal-derived
factors.
The growth factor b-FGF, which is an important endogenous
stimulator of angiogenesis (Montesano et al., 1986)
and cell proliferation (Nissen et al., 1996), is released
from surrounding wounded tissues during an early phase
of wound healing (Cordon-Cardo et al., 1990; Schulze-Osthoff
et al., 1990). Cellular b-FGF is released by the lysis
of epidermal cells (Takamiya et al., 2002), fibroblasts
(Takamiya et al., 2002), and endothelial cells (Muthukrishnan
et al., 1991) around the wound, and b-FGF bound up
in the extracellular matrix is released by the action
of various wound proteases (Bashkin et al., 1989;
Ishai-Michaeli et al, 1990). KGF is expressed in the
dermis during wound healing (Werner et al., 1992),
and stimulates wound reepithelialization (Werner et
al., 1994). HGF was independently discovered as a
powerful mitogen for hepatocytes and as a stimulator
of dissociation of epithelial cells (Werner et al.,
2003). HGF-producing cells are found among those of
mesenchymal origin, and HGF stimulates cell proliferation,
cell migration (Matsumoto et al., 1991), and the production
of matrix metalloproteinase (MMP) (Dunsmore et al.,
1996) in keratinocytes.
Wound healing consists of several stages, including
inflammation, new tissue formation, and tissue remodeling,
eventually leading to at least partial regeneration
of the wounded area (Werner et al., 2003). Various
growth factors directly or indirectly control these
wound-healing phenomena, and can be detected in cutaneous
wound fluids (Grayson et al., 1993; Ono et al, 1995;
Vogt et al., 1998), or in wound fluids obtained through
surgical suction drains (Matsuoka et al., 1989; Baker
et al., 2003; Karayiannakis et al., 2003). The fluid
composition and the concentration of growth factors
in the wound fluids change as healing progresses,
and thus the fluids reflects the sequential phenomena
that occur during wound healing. However, there is
currently only limited information pertaining to the
characterization of surgical drainage fluid with regard
to growth factors and other soluble factors. Although
a few previous reports have suggested that wound fluids
have mitogenic and chemotactic effects (Chen et al.,
1992; Nissen et al., 1996; Trengove et al. 2000),
there has been limited information beyond that. Also,
although it may be clinically feasible to use wound
fluids for cell-based regenerative therapies, there
is limited information on this point, and protocols
for use of wound fluids have yet to be optimized.
The present study focuses on the characterization
of subcutaneous wound fluids obtained through surgical
suction drains. Such fluids are can be aseptically
harvested with minimal morbidity: for example, adipose-derived
stem cells can be isolated from liposuction aspirates
and subcutaneous wound fluids can be simultaneously
obtained by leaving a suction drainage tube in the
subcutaneous cavity in the same surgery. We sequentially
collected surgical drainage fluids from the subcutaneous
space after plastic surgery, and characterized the
fluids by examining wound healing-associated soluble
factors such as electrolytes, cytokines, chemokines
and matrix metalloproteinases (MMPs). We compared
these characterization profiles with those of platelet-poor
and platelet-rich plasma, which can be also easily
obtained from patients, and all three types of fluids
were assessed for their potential utility in cell
culture.
MATERIALS AND METHODS
Collection and preparation of suction
drainage fluid samples
We collected drainage fluids and punctured fluids
from subcutaneous wounds from 21 patients, who underwent
liposuction and abdominoplasty (7 patients), liposuction
(5 patients), breast augmentation (3 patients),
foreign body excision in breasts (2 patients), reduction
mammoplasty (1 patient), cartilage transplantation
for microtia (1 patient), facelift (1 patient) and
parotid gland excision (1 patient) at the University
of Tokyo Hospital. Before samples were obtained,
all patients gave signed informed consent, as approved
by the ethical committee of University of Tokyo
School of Medicine. Suction drains (J-vac drainage
system, Johnson & Johnson, Cornelia, GA, USA)
were subcutaneously inserted into operative wounds
during surgery, after which wound fluid was continuously
suctioned at 40-60 mmHg and aseptically collected
in the storage bag of the suction system. The wound
fluids were centrifuged at 2,000 g for 30 minutes,
and supernatant fluids were frozen at -80°C. The
frozen samples were thawed at 37°C before analysis.
Fifty-two drainage fluid samples were obtained from
18 patients whose wounds exhibited normal healing
(NH group). Nineteen samples harvested on day 0
or day 1 from 15 patients were referred to as Drainage
Fluid-Early (DF-E), while 9 samples harvested on
day 5 or day 6 from seven patients were referred
to as Drainage Fluid-Late (DF-L). In addition, punctured
fluid samples were obtained from 3 patients who
underwent abdominoplasty and had subcutaneous seroma
formation (SF group). These samples were harvested
by puncturing the subcutaneous seroma on day 14
or later, and were included in our analyses as Punctured
fluids (PF).
Collection and preparation of human sera from platelet-rich
plasma and platelet-poor plasma.
Human platelet-rich plasma (PRP) and platelet-poor
plasma (PPP) were prepared from four healthy volunteers.
Blood was drawn into two 200 ml blood bags containing
0.327% citric acid, 2.63% sodium citrate, 0.0275%
adenine, 0.251% sodium dihydrogenphosphate and 2.9%
D-glucose solution (blood bag CPD-adenineR, Terumo,
Tokyo, Japan). To isolate PRP, bags were centrifuged
at 200 g for 10 min in a large-capacity refrigerated
centrifuge (KUBOTA 9810, KUBOTA Co., Tokyo, Japan),
and to isolate PPP the bags were centrifuged at
5000 g for 5 min; in both instances the supernatant
was harvested. PPP was harvested also from 11 of
the aforementioned NH group patients. To obtain
serum from PRP (designated SPRP) and from PPP (designated
SPPP), 100 ml of PRP or PPP was drawn into a flask
and 200 U of thrombin was added. The flask was agitated
for 60 min at 37°C and then incubated overnight
at 4 °C, after which the liquid component was drawn
into 50 ml tube, centrifuged at 2000 g for 10 min,
and supernatants were obtained as for SPRP and SPPP.
The serum samples were frozen at -80°C and thawed
at 37°C before analysis.
Biochemical analysis of serum and drainage fluid
constituents
Preoperative serums and sequential drainage fluids
obtained from three patients either before surgery
or on day 0 to day 6 post-operation were subjected
to biochemical analyses for total protein, albumin,
sodium, potassium, chloride, calcium and iron. Analysis
was performed by SRL, Inc. (Tachikawa, Japan), a
commercial analysis service.
Quantitative assays for cytokines, chemokines, and
matrix metalloproteinases associated with wound
healing
Concentrations of various cytokine growth factors
(PDGF-BB, EGF, TGF-β1, b-FGF, VEGF, HGF, KGF and
IGF-1) and chemokines (IL-6 and IL-8) in drainage
fluid samples, punctured fluids, PRP, and PPP were
assayed using anti-human ELISA kits (QuantikineR,
R&D Systems Inc., Minneapolis, MN), according
to the manufacturer’s instructions. Levels of immunoreactive
cytokines as reported by the ELISA assay were measured
at 450 nm by a microplate reader (Model 550, Biorad
Laboratories, Hercules, CA), and a standard curve
was generated to determine growth factor concentrations
(pg/mL). Levels of three matrix metalloproteinases
(MMP-1, MMMP-8 and MMP-13) in drainage fluids were
also measured using anti-human ELISA kits (Biotrak
ELISA System, Amersham Biosciences, Piscataway,
NJ).
Primary cell culture
Adipose-derived stromal cells (ASCs) were isolated
from human lipoaspirates and cultured as previously
described (Yoshimura et al., 2006). Briefly, the
suctioned fat was digested with 0.075% collagenase
in PBS for 30 min with agitation at 37oC. Mature
adipocytes and connective tissues were separated
from pellets by centrifugation (800 g, 10 min).
Cell pellets were resuspended in erythrocyte lysis
buffer (155mM NH4Cl, 10mM KHCO3, 0.1mM EDTA), incubated
for 5 min at room temperature, resuspended again
and passed through a 100-μm mesh filter (Millipore,
MA, USA), and then plated at a density of 5 x 106
nucleated cells/100 mm plastic dish. Cells were
cultured in M-199 medium containing 10% FBS at 37oC
under 5% CO2 in a humidified incubator.
Human dermal fibroblasts were isolated from normal
skin samples obtained from plastic surgery. The
skin samples were cut into pieces of approximately
3 × 3 mm and treated with 0.25 % trypsin in PBS
for 24 hour at 4°C. After removal of the epidermis,
the connective tissue fragments were attached to
100 mm plastic dishes and cultured with DMEM containing
10% FBS. Primary fibroblasts appeared 4 to 7 days
after the initiation of outgrowth cultures and became
confluent after 2 to 3 weeks.
Cell proliferation assay using culture medium containing
drainage fluids
Culture media (M199 for ASCs and DMEM for dermal
fibroblasts) containing FBS and/or drainage fluids
(DF-E [early] or DF-L [late]) was prepared at various
concentrations. The drainage fluid samples were
sterilized using a 0.22 μm filter (MILLEX GV Filter
Unit, Millipore) before use. 5x104 cells were plated
in 60mm dishes containing the prepared medium, and
the medium was changed on the third and fifth days.
The cell numbers were counted on the seventh day
using a NucleoCounter (Chemometec, Allerod, Denmark),
and average numbers were calculated from three different
cultures of the cell types for each condition. The
averages were normalized by calculating a ratio
of cell numbers grown in each condition to cell
numbers grown in the standard culture conditions
(5% FBS without drainage fluid).
Statistics
The Mann-Whitney U-test with the Bonferroni correction
was used to compare concentrations of cytokines,
chemokines and MMP among drainages fluids (DF-E
and DF-L), punctured fluids, and SPPP. The Mann-Whitney
U-test alone was used for comparison between SPPP
and SPRP and also to compare cell numbers in various
culture conditions. P < 0.05 was considered to
be significant.
RESULTS
Biochemical profiles of drainage
fluids and blood serum
We measured daily changes of electrolytes, albumin,
and total protein in drainage fluids taken from
three patients in the normal healing (NH) group
from day 0 to day 6, and compared these values with
the preoperative serum measurements of these factors
from the same patients (Fig. 1). Concentrations
of total protein and albumin in drainage fluids
on day 0 were about 50% of those in preoperative
serum, and both total protein and albumin gradually
decreased to about 30% of that found in serum by
day 6 (Fig. 1). Concentrations of Na+, K+, Cl-,
Ca++, and Fe++ in drainage fluids were similar to
those in serum and did not significantly change
with time. The concentration of Ca++ in drainage
fluids was about 60-70% of that in serum and changed
very little from day 0 to day 6. The concentration
of Fe++ in drainage fluid was extremely variable
among different patients due to variations in an
individual’s hemorrhage volume, but in general was
substantially greater than that in serum, and tended
to decrease slightly with time.
Cytokine concentrations in drainage fluids, SPRP
and SPPP
Daily sequential changes in various cytokine growth
factors in drainage fluids from six (VEGF, KGF)
or seven (all other cytokines) patients are shown
in Fig. 2, and data from early and late drainage
fluids (DF-E, DF-L), puncture fluid (PF), serum
from platelet-poor plasma (SPPP) and serum from
platelet-rich plasma (SPRP) are summarized in Fig.
3. DF-E and DF-L were harvested from patients in
the NH group, while PF was harvested from patients
in the seroma formation (SF) group. Concentrations
of b-FGF, PDGF, EGF and TGF-β were much higher in
DF-E than in DF-L or SPPP. The concentrations in
DF-E (mean± S.E.) and their relative abundance in
DF-E vs. DF-L were as follows: b-FGF, 678.8 ± 100.6
pg/mL, 34.8x DF-L; EGF, 125.0 ± 18.6 pg/mL, 58.7x
DF-L; PDGF, 267.7 ± 64.2 pg/mL, 72.7x DF-L; and
TGF-β, 45.1 ± 8.4 pg/mL, 9.4x DF-L. In SPRP, B-FGF
was present only at very low levels, while PDGF,
EGF, and TGF-β were abundant, and were significantly
higher in SPRP than in SPPP. Both DF-E and PF contained
high levels of TGF-β, although the concentration
of TGF-β in PF was on average lower than in SPRP.
Concentrations of b-FGF, EGF and PDGF were sufficiently
low in PF that they could not be detected.
In DF-L and in PF, concentrations of VEGF and HGF
were higher than in DF-E and gradually increased
with successive postoperative days, although KGF
concentrations peaked at day 3 and then began to
decrease, in contrast to VEGF and HGF concentrations,
which steadily increased through day 14. PF contained
significantly higher amounts of VEGF, HGF, and KGF
than DF-E, and SPPP and SPRP contained much lower
concentrations of VEGF, HGF, and KGF compared to
DF-E, DF-L, and PF (Fig.3). IGF-1 did not change
significantly with postoperative time, and the concentration
of IGF-1 in DF-E and DF-L was significantly lower
(by approximately 50%) compared to SPPP and SPRP.
Chemokine and MMP concentrations in drainage fluids,
SPRP and SPPP
Daily sequential changes in the IL-6 and IL-8 chemokines
and in MMPs (collagenases) were tracked in drainage
fluids from either five (IL-6, IL-8) or four (MMPs)
patients in the NH group. The summarized data for
levels of these factors in DF-E, DF-L, PF, and SPRP
are shown in Fig. 4 and Fig. 5. IL-6 was present
at high concentrations in DF-E and decreased gradually
in successive postoperative days, while IL-8 increased
gradually. DF-E contained twice as much IL-6 as
was found in DF-L, while DF-L contained twice as
much IL-8 as was found in DF-E. Both IL-6 and IL-8
were detected in small amounts in SPRP, but not
in SPPP, and PF contained both chemokines, although
there were significant differences in PF chemokine
levels vs. chemokine levels in either DF-E or DF-L
(Fig. 4). In drainage fluids, levels of MMP-13 were
low and did not change with time. The concentration
of MMP-1 was also low and though it increased somewhat
with time, it remained relatively slight. In contrast,
MMP-8 increased in the early phase of wound healing,
peaked on day 2-3, and then gradually decreased
with time. In drainage fluids, MMP-8 was present
in much higher amounts as compared to MMP-1 and
MMP-13 (Fig. 5).
Cell proliferation assays using culture medium including
the drainage fluids
In medium that had not been supplemented with drainage
fluids, ASCs proliferated in a dose-dependent manner
with regard to the concentration of FBS; the dose-dependent
relationship was valid up to 10% FBS (Fig. 6A).
When ASCs were grown in media containing 5% FBS,
cell proliferation was significantly enhanced by
the addition of DF-E at concentrations of ?1% or
by the addition of DF-L at concentrations of ?2%.
The increase in proliferation was dose-dependent
with respect to the concentration of DF-E or DF-L:
When 5% FBS and 5% DF-E were added to the medium,
cell count increased to over five times that of
the control (5% FBS alone), and was twice as high
as the cell count for media containing 10% FBS alone.
In medium lacking FBS, the addition of drainage
fluids significantly enhanced ASC proliferation
but were less effective compared to the same concentrations
of FBS. We also examined dermal fibroblasts, which
proliferated in a dose-dependent manner with respect
to FBS up to concentrations of 10% FBS (Fig. 6B).
The proliferation of dermal fibroblasts was enhanced
by drainage fluids in the absence of FBS, though
the enhancement of proliferation by the addition
of drainage fluids to 5% FBS was not so striking
compared to that of the ASCs. Table 1 shows summary
data for the concentrations of growth factors, cytokines,
and MMPs in DF-E, DF-L, SPPP, and SPRP. We suggest
that this data could be used as a guide in choosing
the appropriate fluid supplement for cell culture,
based on the specific needs of a given cell line.
Comparison to previous studies
We compared our data on drainage fluids from subcutaneous
wounds with data from previous studies on fluids
from peritoneal drainages (Baker et al., 2003) and
on wound fluids from split-thickness skin donor
sites (Vogt et al., 1998) (Fig. 7). We found that
the source of the wound fluid affected cytokine
growth factor profiles: In general, skin wound fluids
contained substantially lower levels of growth factors
(bFGF, EGF, and TGF-β) compared to subcutaneous
or peritoneal drainage fluids. Peritoneal drainage
fluids contained over 100 times more TGF-β compared
to subcutaneous and skin wound drainage fluids.
Subcutaneous and peritoneal drainage fluids similarly
contained higher concentrations of b-FGF, EGF, and
VEGF. The difference in the cytokine profiles may
reflect the requirement for different biochemical
conditions for the optimal healing of each wound
type.
DISCUSSION
Biochemical analysis of drainage fluids
Results from the analysis of the biochemical composition
of drainage fluids in this study were similar to
those of a previous study analyzing biochemical
profiles of drainage fluids after axillary dissection
(Bonnema et al., 1999). Our analysis showed that
concentrations of Na+, K+, and Cl- in drainage fluids
were similar to those in plasma, while concentrations
of Ca++, total protein and albumin in drainage fluids
were ~60-80% lower than those in plasma. The concentration
of Fe++ in drainage fluid was generally higher than
in plasma but was variable, (depending on the hemorrhage
volume) and decreased over time.
Extracellular fluid volume, which makes up approximately
20% of body weight, is composed of 5% plasma (or
intravascular fluid) and 15% interstitial fluid.
The concentrations of Na+, K+, and Cl- in interstitial
fluid are similar to those in plasma, while the
total protein concentration of interstitial fluid
is less than a third of that of plasma (Wait et
al., 2001). It is therefore likely that our drainage
fluids consisted primarily of interstitial fluid,
with plasma composing the remaining ~20-40% of the
total volume. However, drainage fluids are not simply
a mixture of plasma and interstitial fluids because
unlike these fluids, they also contain several other
types of proteins, including various cytokine growth
factors and chemokines.
Cytokine growth factor profiles in drainage fluids
The sequential changes in cytokine profiles in drainage
fluids shown in this study clearly reflected the
successive activities of various cells and the sequential
phenomena involved in the wound healing process.
Fig. 8 summarizes the sequential changes in the
profiles of cytokines, chemokines, and MMPs, as
measured in this study. In the early phase (postoperative
days 0-1) of wound healing, b-FGF, PDGF, EGF, and
TGF-β1 were present at high concentrations, and
levels subsequently decreased acutely in the next
stage of wound healing. Since b-FGF is known to
be primarily derived from injured tissue or from
cells infiltrating into wounds at early stages,
tissue-bound b-FGF may be released after injury
by several mechanisms, including cell lysis and
cell injury (Muthukrishnan et al., 1991; Baker et
al., 2000; Takamiya et al., 2002). PDGF, EGF, and
TGF-β1 were detected at higher amounts in SPRP than
in DF-E, suggesting that these growth factors were
mainly supplied by dying, lytic platelets in the
early phase of wound healing.
In the second phase of wound healing (postoperative
days 2-4), KFG concentrations peaked, and those
VEGF and HGF slightly increased. Later, in the third
phase, (days 5-6) VEGF and HGF concentrations gradually
increased to peak levels. Since these growth factors
were present at only very low levels on days 0-1,
it is possible that these increases resulted from
their release from the cells that migrated to the
wound site after day 1. KFG, VEGF, and HGF are thought
to have roles primarily in granulation, angiogenesis
and epithelialization (Peters et al., 1993; Gale
et al., 1999; Lauer et al., 2000). KGF is known
to be released mainly from fibroblasts and T cells
(Brauchle et al., 1994; Marchese et al. 1995), VEGF
from keratinocytes and macrophages (Brown et al.,
1992; Frank et al. 1995), and HGF from mesenchymal
cells such as dermal fibroblasts (Matsumoto et al.,
1991).
Punctured fluids from subcutaneous seroma contained
higher concentrations of growth factors seen in
later phases such as VEGF, HGF, and KGF, but TGF-β1,
which is seen in the early phase, was also abundant
in seroma fluid. This finding may be based on different
sources of the early-phase growth factors: PDGF
and EGF are mostly derived from platelet, whereas
TGF-β1 is supplied not only from platelets but also
from various sources. The initial production of
active TGf-β1 from platelets serves as a chemoattractant
for neutrophils, macrophages, and fibroblasts, and
these cells further enhance TGF-β1 production (Werner
et al., 2002). As well as active forms, lent TGF-βs
are also produced and sequestered within the wound
matrix, allowing its sustained release by proteolytic
enzymes (Gailit et al., 1994; Zambruno et al., 1995),
and the combination of different cellular sources
and temporary storage ensures a continuous supply
of TGF-β1 throughout the repair process (Werner
et al., 2002). A study using knock-out mice of TGF-β1
(Brown et al., 1995) showed that different growth
factors can compensate for the lack of TGF-β1 in
early wounds, but that TGF-β1 plays a crucial role
later in the repair process.
Chemokine and MMP profiles in drainage fluids
We also examined IL-6 and IL-8, which are two pro-inflammatory
chemokines (chemotactic cytokines). IL-6 is a major
mediator of the host response to tissue injury (Sehgal,
1990), and is released by a variety of cell types
including polymorphonuclear leukocytes, macrophages,
fibroblasts, lymphocytes and endothelial cells (Panquet
et al., 1996). IL-8 has been shown to stimulate
chemotaxis of neutrophils (Hoch et al., 1996) and
keratinocytes (Kemeny et al. 1994) and also to stimulate
neovascularization (Koch et al., 1992). In our study,
IL-6 was present in DF-E at about 7000 pg/mL and
gradually decreased afterwards. Cells that appeared
in the wound area in each phase seemed to be major
sources of IL-6; neutrophils were present in the
early phase and macrophages and lymphocytes were
present in later phases (Fig. 8). In the case of
IL-8, the concentration gradually increased up to
day 6, so it is probable that the fibroblasts present
in the second wave of cell migration to the wound
produced significant amounts of IL-8, as was previously
suggested in a study of fetal wound healing (Liechty
et al., 1998).
Pro-inflammatory cytokines/chemokines directly stimulate
the synthesis of the collagen-degrading matrix metalloproteinases
(MMPs) and also inhibit the synthesis of tissue
inhibitors of metalloproteinase in fibroblasts and
endothelial cells (Murphy et al., 1994). We measured
three types of collagenases, MMP-1, MMP-8 and MMP-13.
MMP-1 preferentially degrades type III collagen
(Welgus et al., 1981), whereas MMP-8 has its greatest
activity on type I collagen (Hasty et al., 1987).
MMP-13 can degrade all fibrillar collagen subtypes
with nearly equal efficacy and is the only collagenase
with significant activity against type II and IV
collagen (Freije et al., 1994). Fibroblasts appear
to be the cellular source for the majority of MMP-1,
while neutrophils seem to provide most of MMP-8.
In subcutaneous drainage fluids, MMP-1 gradually
increased up to day 6, while MMP-8 peaked on days
2 to 3. At all time points examined, levels of MMP-8
were statistically significantly higher than both
MMP-1 (50-fold to 200-fold) and MMP-13 (1000-fold
to 10000 fold). The sequential changes that we observed
in MMP-1 and MMP-8 were similar to previously reported
data from a study of cutaneous wound fluids (Nwomeh
et al., 1998). Taken together, these data suggest
that MMP-8 functions as the primary debriding collagenase
during the acute phase of wound healing.
Potential use of wound fluids and SPRP in culture
media
Since our data showed that drainage fluids contained
various growth factors which were not found in SPPP
or SPRP, we tested drainage fluids as a substitute
or supplement for serum in the culturing of ASCs
and dermal fibroblasts. The experiment using ASCs
showed that DF-E is superior to FBS as a 5% additive
of the medium containing 5% FBS, while that using
dermal fibroblasts suggested that drainage fluids
may be used as similarly to serum. Thus, we suggest
that drainage fluids may be used as a supplement
or substitute for serum in culture media, and may
be able to support the growth of cell types other
than the two lines examined in this study.
Recent developments in the clinical use of cultured
cells (such as stem cell therapy or gene delivery)
have necessitated safer preparation and manipulation
of cells, which partly entails avoiding the use
of animal-derived serum, tissues and extracts. In
this respect, autologous serum, cytokines or other
soluble factors could be extremely valuable. For
example, ASCs isolated from liposuction aspirates
of a patient could be cultured using the patient’s
own SPRP taken from blood and/or using drainage
fluids taken from the subcutaneous wound after liposuction.
Subcutaneous wound fluids have some advantages compared
to cutaneous and intraperitoneal wound fluids: subcutaneous
wound fluids are difficult to collected aseptically
and in a large volume, and intraperitoneal ones
can be obtained only through major abdominal surgery
and are not aseptic in most cases.
Our results have shown that SPRP and drainage fluids
can be abundant sources of autologous soluble factors
such as cytokines, and our biochemical breakdown
of DF-E, DF-L, SPPP, and SPRP provides the ability
to assess the culture requirements of a particular
cell line and then to select an appropriate fluid
for use as a supplement or substitute for FBS in
cell culture. Both SPRP and drainage fluids are
economical ready-made mixtures of serum (plasma)
and soluble factors such as cytokines, and can also
be used as raw materials for the extraction of individual
soluble factor proteins. Further investigation will
be necessary to provide optimized protocols for
the usage of drainage fluids and SPRP in cell culture
and in factor isolation.
Figure Legends
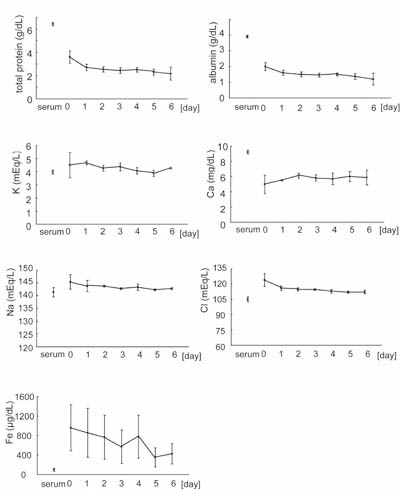
Fig. 1
Biochemical profiles of preoperative serum and of
drainage fluids on days 0 to 6.
Preoperative serum and drainage fluids were collected
from the same three patients; drainage fluids were
collected on days 0-6, where day 0 represents the
day that surgery was performed. The preoperative
serum value is indicated to the left on the x-axis
and is labeled “serum”; the numbers 0-6 to the right
represent day 0 through day 6 timepoints of drainage
fluid collection. Values represent means ± S.E.
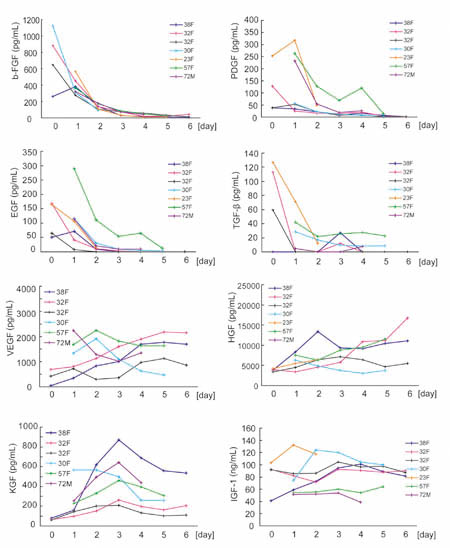
Fig. 2
Daily changes in cytokine concentrations in drainage
fluids.
Drainage fluids were collected on days 0 to 6 from
patients in the normal healing (NH) group, and cytokine
concentrations were examined by ELISA. For VEGF
and KGF, data was derived from six patients; for
all other cytokines, data was derived from seven
patients Each line shows data from one patient (e.g.
38F means 38 year-old female).
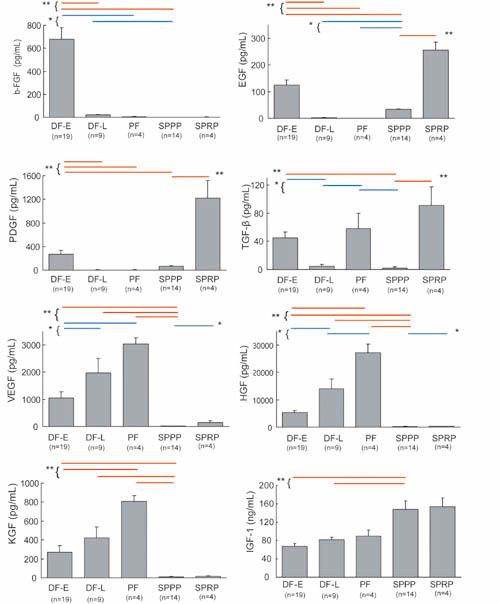
Fig. 3
Comparison of cytokine concentrations in DF-E, DF-L,
PF, SPPP, and SPRP.
Cytokine concentrations were measured in drainage
fluid samples from day 0 or day 1 (DF-E; Drainage
Fluid-Early), drainage fluid from day 5 or day 6
(DF-L; Drainage Fluid-Late), punctured seroma fluid
samples from days 14+ (PF; Punctured Fluids), serum
from platelet-poor plasma (SPPP), and serum from
platelet-rich plasma (SPRP). Cytokine concentrations
in DF-E, DF-L, and PF were compared to those of
SPPP and SPRP. Values represent means ± S.E. * P<0.05
(blue lines), ** P<0.01 (red lines).
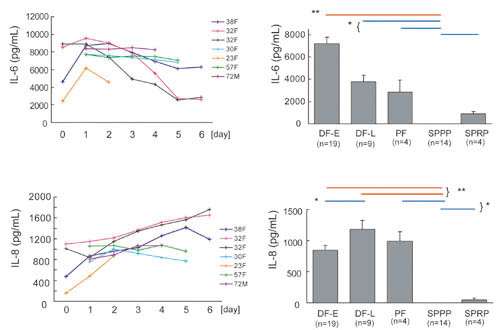
Fig. 4
Changes in IL-6 and IL-8 concentrations in drainage
fluids and comparison to PF, SPPP and SPRP.
The concentration of IL-6 and IL-8 was measured
by ELISA in drainage fluids collected on days 0-6
and was similarly measured in PF, SPPP, and SPRP.
Data of daily changes from seven patients were shown
in left figures, in which each line shows data from
one patient (e.g. 38F means 38 year-old female).
Values in right graphs represent means ± S.E. *
P<0.05 (blue lines), ** P<0.01 (red lines).
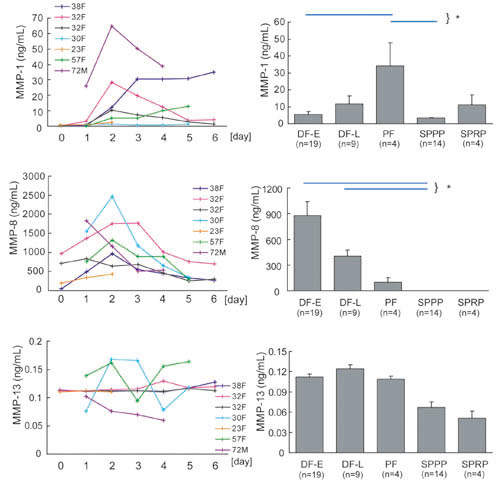
Fig. 5
Changes in concentrations of MMP-1, MMP-8, and MMP-13
in drainage fluids and comparison to PF, SPPP and
SPRP.
The concentration of MMP-1, MMP-8, and MMP-13 was
measured by ELISA in drainage fluid collected on
days 0-6 and in seroma puncture fluid (PF), SPPP,
and SPRP. Data of daily changes from seven patients
were shown in left figures, in which each line shows
data from one patient (e.g. 38F means 38 year-old
female). Values in right graphs represent means
± S.E. * P<0.05 (blue lines).
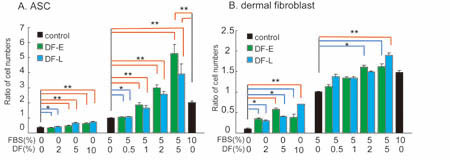
Fig. 6
Proliferation of ASCs and dermal fibroblasts.
ASCs (A) and dermal fibroblasts (B) were cultured
with DMEM containing various amounts of FBS and/or
drainage fluids (DF-E or DF-L) for 1 week and cell
numbers were counted. Each cell number was expressed
as a ratio to that of the control culture, which
was grown in media that contained 5% FBS and lacked
drainage fluid. Values represent means ± S.E. *
P<0.05 (bracketed blue lines), ** P<0.01 (bracketed
red lines).
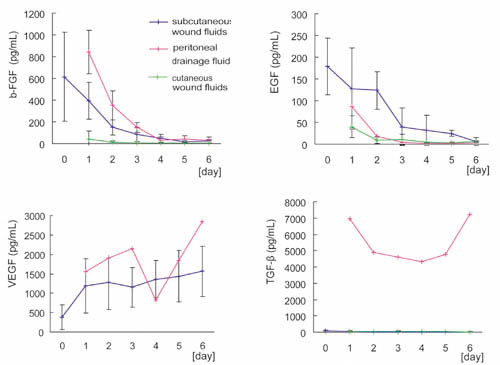
Fig. 7
Comparison of cytokine concentrations with data
of previous studies.
Data from this study on cytokine concentrations
in drainage fluids from subcutaneous wounds were
compared with data from two previous studies examining
drainage fluids from intraperitoneal wounds (Baker
et al., 2003) and fluids from cutaneous wounds;
the donor sites of split skin grafts (Vogt et al.,
1998). Values represent means ± S.D.
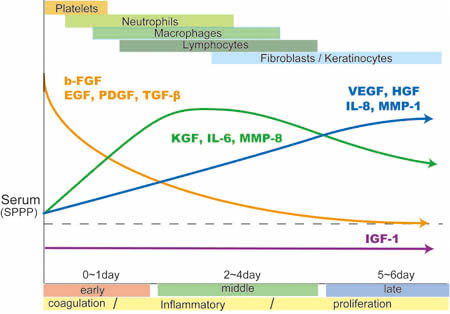
Fig. 8
Summary of sequential changes in soluble factors
associated with wound healing in drainage fluids
from subcutaneous wounds.
There are three types of sequential changes in the
abundance of soluble factors that function in wound
healing. First, levels of b-FGF, EGF, PDGF and TGF-β
are initially high and then gradually decrease.
EGF and PDGF in drainage fluids in the early phase
(coagulation phase) of wound healing are derived
from platelets, although TGF-β is derived from various
sources, and b-FGF is mainly derived from injured
tissue or from cells infiltrating into wounds at
early stages. Second, KGF, IL-6, and MMP-8 peak
around days 2 to 4 (during the inflammatory phase).
KGF is released from T lymphocytes and fibroblasts,
while IL-6 seems to be discharged from the various
cells involved in each phase. Third, VEGF, HGF,
IL-8, and MMP-1 are low in the early phase and gradually
increase up to the late phase (proliferation phase).
These factors are derived from cells involved in
the later phases of wound healing, including fibroblasts
and keratinocytes. IGF-1 is present at relatively
consistent levels throughout the entire wound healing
process. MMP-13 is detected only in minimal quantities.
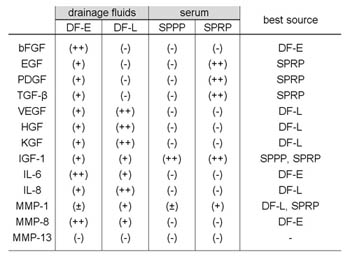
Table 1
Sources of autologous soluble factors associated
with wound healing: comparison of drainage fluids,
SPPP and SPRP.
Relative abundances are indicated by -, +/-, +,
and ++. ++ indicates high abundance of a factor,
and ? indicates absence of a factor.
References
Baker EA, Gaddal SE, Aitken DG,
Leaper DJ. 2003. Growth factor profiles in intraperitoneal
drainage fluid following colorectal surgery: relationship
to wound healing and surgery. Wound Repair Regen
11: 261-267.
Baker EA, Leaper DJ. 2000. Proteinases,
their inhibitors, and cytokine profiles in acute
wound fluid. Wound Repair Regen 8: 392-398.
Bashkin P, Doctrow S, Klagsbrun
M, Svahn CM, Folkman J, Vlodavsky I. 1989. Basic
fibroblast growth factor binds to subendothelial
extracellular matrix and is released by heparitinase
and heparin-like molecules. Biochemistry 28: 1737-1743.
Bonnema J, Ligtenstein DA, Wiggers
T, van Geel AN. 1999. The composition of serous
fluid after axillary dissection. Eur J Surg 165:
9-13.
Brauchle M, Angermeyer K, Hubner
G, Werner S. 1994. Large induction of keratinocyte
growth factor expression by serum growth factors
and pro-inflammatory cytokines in cultured fibroblasts.
Oncogene 9: 3199-3204.
Brown LF, Yeo KT, Berse B, Yeo
TK, Senger DR, Dvorak HF, van de Water L. 1992.
Expression of vascular permeability factor (vascular
endothelial growth factor) by epidermal keratinocytes
during wound healing. J Exp Med 176: 1375-1379.
Brown RL, Ormsby I, Doetschman
TC, Greenhalgh DG. 1995. Wound healing in the transforming
growth factor-β1-deficient mouse. Wound Repair Regen
3: 25-36.
Chen WY, Rogers AA, Lydon MJ. 1992.
Characterization of biologic properties of wound
fluid collected during early stages of wound healing.
J Invest Dermatol 99: 559-564.
Cordon-Cardo C, Vlodavsky I, Haimovitz-Friedman
A, Hicklin D, Fuks Z. 1990. Expression of basic
fibroblast growth factor in normal human tissues.
Lab Invest 63: 832-840.
Dunsmore SE, Rubin JS, Kovacs SO,
Chedid M, Parks WC, Welgus HG. 1996. Mechanisms
of hepatocyte growth factor stimulation of keratinocyte
metalloproteinase production. J Biol Chem 271: 24576-24582.
Eppley BL, Woodell JE, Higgins
J. 2004. Platelet quantification and growth factor
analysis from platelet-rich plasma: implications
for wound healing. Plast Reconstr Surg 114: 1502-1508.
Frank S, Hubner G, Breier G, Longaker
MT, Greenhalgh DG, Werner S. 1995. Regulation of
vascular endothelial growth factor expression in
cultured keratinocytes. Implications for normal
and impaired wound healing. J Biol Chem 270:12607-12613.
Freije JM, Diez-Itza I, Balbin
M, Sanchez LM, Blasco R, Tolivia J, Lopez-Otin C.
1994. Molecular cloning and expression of collagenase-3,
a novel human matrix metalloproteinase produced
by breast carcinomas. J Biol Chem 269: 16766-16773.
Gale NW, Yancopoulos GD. 1999.
Growth factors acting via endothelial cell-specific
receptor tyrosine kinases: VEGFs, angiopoietins,
and ephrins in vascular development. Genes Dev 13:1055-1066.
Grayson LS, Hansbrough JF, Zapata-Sirvent
RL, Dore CA, Morgan JL, Nicolson MA. 1993. Quantitation
of cytokine levels in skin graft donor site wound
fluid. Burns 19: 401-405.
Hasty KA, Jeffrey JJ, Hibbs MS,
Welgus HG. 1987. The collagen substrate specificity
of human neutrophil collagenase. J Biol Chem 262:
10048-10052.
Hoch RC, Schraufstatter IU, Cochrane
CG. 1996. In vivo, in vitro, and molecular aspects
of interleukin-8 and the interleukin-8 receptors.
J Lab Clin Med 128: 134-145.
Ishai-Michaeli R, Eldor A, Vlodavsky
I. 1990. Heparanase activity expressed by platelets,
neutrophils, and lymphoma cells releases active
fibroblast growth factor from extracellular matrix.
Cell Regul.1: 833-42.
Karayiannakis AJ, Zbar A, Polychronidis
A, Simopoulos C. 2003. Serum and drainage fluid
vascular endothelial growth factor levels in early
surgical wounds. Eur Surg Res 35: 492-496.
Kemeny L, Ruzicka T, Dobozy A,
Michel G. 1994. Role of interleukin-8 receptor in
skin. Int Arch Allergy Immunol 104: 317-322.
Koch AE, Polverini PJ, Kunkel SL,
Harlow LA, DiPietro LA, Elner VM, Elner SG, Strieter
RM. 1992. Interleukin-8 as a macrophage-derived
mediator of angiogenesis. Science. 258: 1798-1801.
Lauer G, Sollberg S, Cole M, Flamme
I, Sturzebecher J, Mann K, Krieg T, Eming SA. 2000.
Expression and proteolysis of vascular endothelial
growth factor is increased in chronic wounds. J
Invest Dermatol 115:12-18.
Liechty KW, Crombleholme TM, Cass
DL, Martin B, Adzick NS. 1998. Diminished interleukin-8
(IL-8) production in the fetal wound healing response.
J Surg Res 77: 80-84.
Lucarelli E, Beccheroni A, Donati
D, Sangiorgi L, Cenacchi A, Del Vento AM, Meotti
C, Bertoja AZ, Giardino R, Fornasari PM, Mercuri
M, Picci P. 2003. Platelet-derived growth factors
enhance proliferation of human stromal stem cells.
Biomaterials 24: 3095-3100.
Marchese C, Chedid M, Dirsch OR,
Csaky KG, Santanelli F, Latini C, LaRochelle WJ,
Torrisi MR, Aaronson SA. 1995. Modulation of keratinocyte
growth factor and its receptor in reepithelializing
human skin. J Exp Med 182: 1369-1376.
Matsumoto K, Hashimoto K, Yoshikawa
K, Nakamura T. 1991. Marked stimulation of growth
and motility of human keratinocytes by hepatocyte
growth factor. Exp Cell Res 196: 114-20.
Matsuoka J, Grotendorst GR. 1989.
Two peptides related to platelet-derived growth
factor are present in human wound fluid. Proc Natl
Acad Sci USA 86: 4416-4420.
McAlinden MG, Wilson DJ. 2000.
Comparison of cancellous bone-derived cell proliferation
in autologous human and fetal bovine serum. Cell
Transplant 9: 445-451.
Montesano R, Vassalli JD, Baird
A, Guillemin R, Orci L. 1986. Basic fibroblast growth
factor induces angiogenesis in vitro. Proc Natl
Acad Sci USA 83: 7297-7301.
Murphy G, Willenbrock F, Crabbe
T, O'Shea M, Ward R, Atkinson S, O'Connell J, Docherty
A. 1994. Regulation of matrix metalloproteinase
activity. Ann N Y Acad Sci. 732: 31-41.
Muthukrishnan L, Warder E, McNeil
PL. 1991. Basic fibroblast growth factor is efficiently
released from a cytolsolic storage site through
plasma membrane disruptions of endothelial cells.
J Cell Physiol 148: 1-16.
Nissen NN, Polverini PJ, Gamelli
RL, DiPietro LA. 1996. Basic fibroblast growth factor
mediates angiogenic activity in early surgical wounds.
Surgery 119: 457-465.
Nwomeh BC, Liang HX, Diegelmann
RF, Cohen IK, Yager DR. 1998. Dynamics of the matrix
metalloproteinases MMP-1 and MMP-8 in acute open
human dermal wounds. Wound Repair Regen 6: 127-134.
Ono I, Gunji H, Zhang JZ, Maruyama
K, Kaneko F. 1995. Studies on cytokines related
to wound healing in donor site wound fluid. J Dermatol
Sci 10: 241-245.
Paquet P, Pierard GE. 1996. Interleukin-6
and the skin. Int Arch Allergy Immunol 109: 308-17.
Peters KG, De Vries C, Williams
LT. 1993. Vascular endothelial growth factor receptor
expression during embryogenesis and tissue repair
suggests a role in endothelial ifferentiation and
blood vessel growth. Proc Natl Acad Sci USA 90:
8915-8919.
Ross R, Glomset J, Kariya B, Harker
L. 1974. A platelet-dependent serum factor that
stimulates the proliferation of arterial smooth
muscle cells in vitro. Proc Natl Acad Sci USA 71:
1207-1210.
Sanchez AR, Sheridan PJ, Kupp LI.
2003. Is platelet-rich plasma the perfect enhancement
factor? A current review. Int J Oral Maxillofac
Implants 18: 93-103.
Schulze-Osthoff K, Risau W, Vollmer
E, Sorg C. 1990. In situ detection of basic fibroblast
growth factor by highly specific antibodies. Am
J Pathol 137: 85-92.
Sehgal PB. 1990. Interleukin-6:
molecular pathophysiology. J Invest Dermatol 94(Suppl):
2S-6S.
Takimiya M, 2002 Immunohistochemical
Study of Basic Fibroblast Growth Facto and Vasucular
Endothelial Growth Factor Expression for Age Determination
of Cutaneous Wound. The American J of Forensic Medicine
and Pathology. 23(3) 264-267)
Thor A, Wannfors K, Sennerby L,
Rasmusson L. 2005. Reconstruction of the severely
resorbed maxilla with autogenous bone, platelet-rich
plasma, and implants: 1-year results of a controlled
prospective 5-year study. Clin Implant Dent Relat
Res 7: 209-220.
Trengove NJ, Bielefeldt-Ohmann
H, Stacey MC. 2000. Mitogenic activity and cytokine
levels in non-healing and healing chronic leg ulcers.
Wound Repair Regen 8:13-25.
Vogt PM, Lehnhardt M, Wagner D,
Jansen V, Krieg M, Steinau HU. 1998. Determination
of endogenous growth factors in human wound fluid:
temporal presence and profiles of secretion. Plast
Reconstr Surg 102: 117-123.
Wait RB, Kim UK, Mustafa IA. 2001
Fluids, electrolytes, and acid-base balance. In:
Greenfield LJ, editor. Surgery (third edidtion),
Philadelphia,: Lippincott Williams & Wilkins,
p 244-269.
Welgus HG, Jeffrey JJ, Eisen AZ.
1981. The collagen substrate specificity of human
skin fibroblast collagenase. J Biol Chem 256:9511-9515.
Werner S, Peters KG, Longaker MT,
Fuller-Pace F, Banda MJ, Williams LT. 1992. Large
induction of keratinocyte growth factor expression
in the dermis during wound healing. Proc Natl Acad
Sci USA 89: 6896-6900.
Werner S, Smola H, Liao X, Longaker
MT, Krieg T, Hofschneider PH, Williams LT. 1994.
The function of KGF in morphogenesis of epithelium
and reepithelialization of wounds. Science 266:
819-22.
Werner S, Grose R. 2003. Regulation
of wound healing by growth factors and cytokines.
Physiol Rev 83: 835-870.
Yoshimura K, Shigeura T, Matsumoto
D, Sato T, Takaki Y, Aiba-Kojima E, Sato K, Inoue
K, Nagase T, Koshima I, Gonda K. 2006. Characterization
of freshly isolated and cultured cells derived from
the fatty and fluid portions of liposuction aspirates.
J Cell Physiol 208: 64-76.